Synthesis, Characterization, and Self-Assembly of Porphyrins
Total Page:16
File Type:pdf, Size:1020Kb
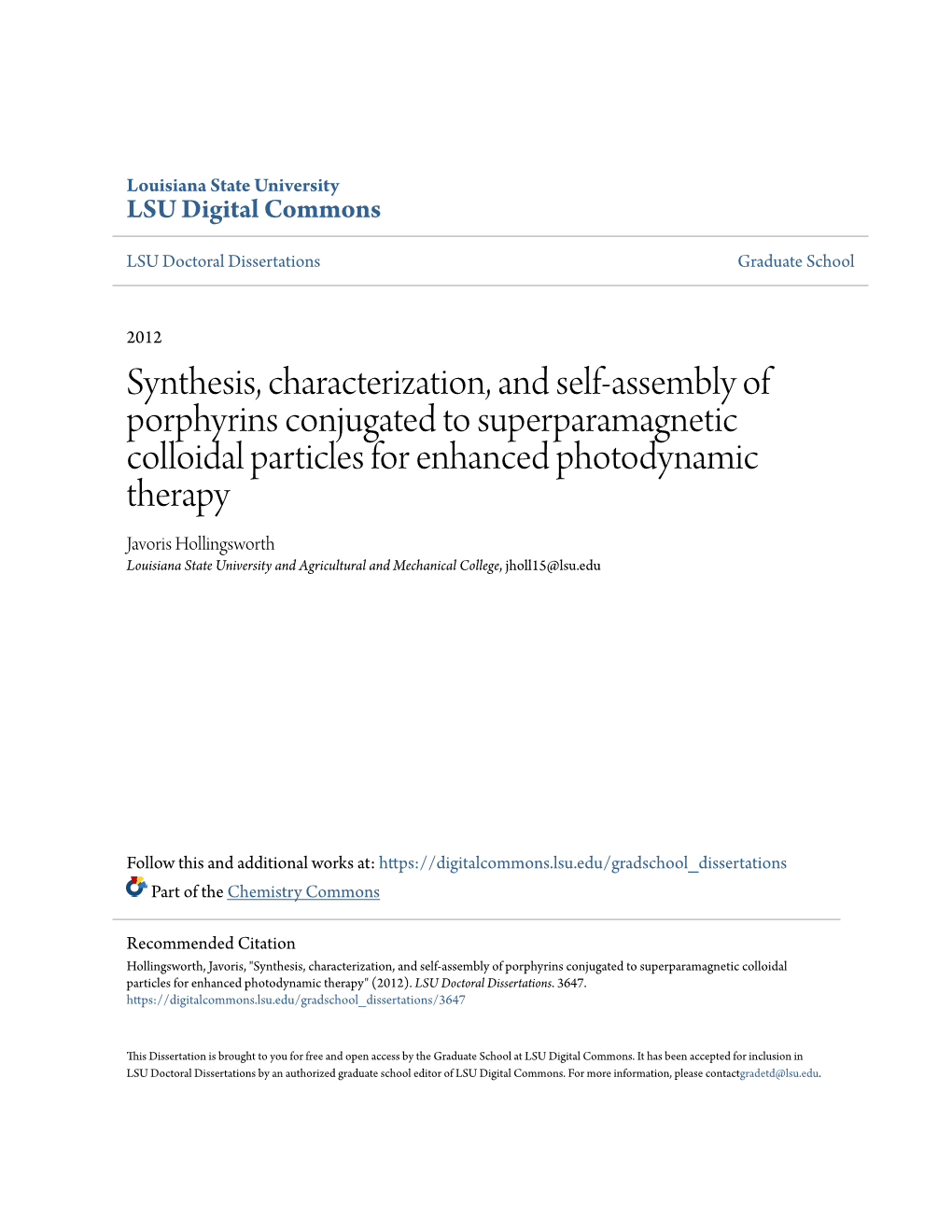
Load more
Recommended publications
-
Photoacoustic Measurements of Porphyrin Triplet-State Quantum Yields and Singlet-Oxygen Efficiencies
FULL PAPER Photoacoustic Measurements of Porphyrin Triplet-State Quantum Yields and Singlet-Oxygen Efficiencies Marta Pineiro, Ana L. Carvalho, Mariette M. Pereira, A. M. dA. Rocha Gonsalves, Luís G. Arnaut,* and SebastiaÄo J. Formosinho Abstract: Photoacoustic calorimetry was used to measure the quantum yields of singlet molecular oxygen production by the triplet states of tetraphenylporphyrin (TPP), ZnTPP and CuTPP in toluene, yielding values of 0.67 Æ 0.14, 0.68 Æ 0.19 Keywords: metalloporphyrins ´ and 0.03 Æ 0.01, respectively. We show that a novel dichlorophenyl derivative of photoacoustic calorimetry ´ por- ZnTPP is capable of singlet-oxygen production with a 0.90 Æ 0.07 quantum yield. phyrinoids ´ singlet oxygen ´ sensi- The synthesis and characterisation of a new photostable chlorin with high absorptivity tizers in the red that is capable of singlet-oxygen production with 0.54 Æ 0.06 quantum yield is described. Our results suggest that chlorinated chlorins may be interesting new sensitisers for photodynamic therapy. Introduction Type II photooxygenation process in cells.[7, 8] Adequate sensitisers have specific biological and photochemical proper- The ubiquitous tetrapyrrolic macrocycles play highly diverse ties. The desired biological features of the sensitiser are: roles in biological systems.[1±5] The natural roles of these 1) little or no dark toxicity structures stimulated the search for new applications, exploit- 2) selective accumulation and prolonged retention in tumour ing in particular the use of new synthetic porphyrins.[6] One of tissues the more recent and promising applications of porphyrin 3) controlled photofading to reduce the unwanted skin chemistry in medicine is in the detection and cure of photosensitivity side effects and increase light penetration tumours,[7, 8] referred to as photodynamic therapy (PDT). -
Electronic Spectroscopy of Free Base Porphyrins and Metalloporphyrins
Absorption and Fluorescence Spectroscopy of Tetraphenylporphyrin§ and Metallo-Tetraphenylporphyrin Introduction The word porphyrin is derived from the Greek porphura meaning purple, and all porphyrins are intensely coloured1. Porphyrins comprise an important class of molecules that serve nature in a variety of ways. The Metalloporphyrin ring is found in a variety of important biological system where it is the active component of the system or in some ways intimately connected with the activity of the system. Many of these porphyrins synthesized are the basic structure of biological porphyrins which are the active sites of numerous proteins, whose functions range from oxygen transfer and storage (hemoglobin and myoglobin) to electron transfer (cytochrome c, cytochrome oxidase) to energy conversion (chlorophyll). They also have been proven to be efficient sensitizers and catalyst in a number of chemical and photochemical processes especially photodynamic therapy (PDT). The diversity of their functions is due in part to the variety of metals that bind in the “pocket” of the porphyrin ring system (Fig. 1). Figure 1. Metallated Tetraphenylporphyrin Upon metalation the porphyrin ring system deprotonates, forming a dianionic ligand (Fig. 2). The metal ions behave as Lewis acids, accepting lone pairs of electrons ________________________________ § We all need to thank Jay Stephens for synthesizing the H2TPP 2 from the dianionic porphyrin ligand. Unlike most transition metal complexes, their color is due to absorption(s) within the porphyrin ligand involving the excitation of electrons from π to π* porphyrin ring orbitals. Figure 2. Synthesis of Zn(TPP) The electronic absorption spectrum of a typical porphyrin consists of a strong transition to the second excited state (S0 S2) at about 400 nm (the Soret or B band) and a weak transition to the first excited state (S0 S1) at about 550 nm (the Q band). -
Design of Porphyrin Solids
DESIGN OF PORPHYRIN SOLIDS ZN···NO2 RECOGNITION, MULTI-STEP SINGLE CRYSTAL TO SINGLE CRYSTAL TRANSFORMATIONS AND COFACIAL DIMERS By SALIMGEREY ADILOV A Dissertation Submitted to the Faculty of the WORCESTER POLYTECHNIC INSTITUTE In partial fulfillment of the requirements for the Degree of Doctor of Philosophy in Chemistry by July 2008 APPROVED: Dr. Venkat R. Thalladi Dr. Dhandapani Venkataraman Advisor External Reviewer; UMass Amherst Dr. James P. Dittami Dr. Weiyong Yu Committee Member Committee Member Dr. Kristin N. Wobbe Head of Department ACKNOWLEDGEMENTS I would like to thank my research advisor Professor Venkat R. Thalladi for his scientific supervision as well as moral support during my stay at WPI. He has always been inspiring and his scientific discussions have been highly encouraging. I learnt a lot from him about science and about life. I would like to thank all the faculty and staff of the Department of Chemistry and Biochemistry at WPI. Thanks to my graduate committee Professors James P. Dittami, Weiyong Yu and Dhandapani Venkataraman. I would like to thank Professor John MacDonald for his discussions throughout my Ph.D. career. Special thanks to Dr. Richard Staples and Dr. Jingwei Huang of Harvard University and Dr. Hans-Christoph Weiss of Bayer, Germany and Professor Roland Bose of University Duisburg-Essen for collecting single crystal X-ray data on some of the structures reported in this thesis. I would like to thank my friendly and supportive labmates. In particular, I thank Marta Dabros, Jason R. Cox, Taner Gokcen and Paul Emery for their help, critical reading and valuable discussions that helped my thesis look better. -
On Tuning the Fluorescence Emission of Porphyrin Free Bases Bonded to the Pore Walls of Organo-Modified Silica
Molecules 2014, 19, 2261-2285; doi:10.3390/molecules19022261 OPEN ACCESS molecules ISSN 1420-3049 www.mdpi.com/journal/molecules Article On Tuning the Fluorescence Emission of Porphyrin Free Bases Bonded to the Pore Walls of Organo-Modified Silica Rosa I. Y. Quiroz-Segoviano 1, Iris N. Serratos 1, Fernando Rojas-González 1, Salvador R. Tello-Solís 1, Rebeca Sosa-Fonseca 2, Obdulia Medina-Juárez 1, Carmina Menchaca-Campos 3 and Miguel A. García-Sánchez 1,* 1 Departamento de Química, Universidad Autónoma Metropolitana-Iztapalapa, Av. San Rafael Atlixco 186, Vicentina, D. F. 09340, Mexico 2 Departamento de Física, Universidad Autónoma Metropolitana-Iztapalapa, Av. San Rafael Atlixco 186, Vicentina, D. F. 09340, Mexico 3 Centro de Investigación en Ingeniería y Ciencias Aplicadas, UAEM, Av. Universidad 1001, Col. Chamilpa, C.P. 62209, Cuernavaca Mor., Mexico * Author to whom correspondence should be addressed; E-Mail: [email protected]; Tel.: +52-55-5804-4677; Fax: +52-55-5804-4666. Received: 24 December 2013; in revised form: 29 January 2014 / Accepted: 7 February 2014 / Published: 21 February 2014 Abstract: A sol-gel methodology has been duly developed in order to perform a controlled covalent coupling of tetrapyrrole macrocycles (e.g., porphyrins, phthalocyanines, naphthalocyanines, chlorophyll, etc.) to the pores of metal oxide networks. The resulting absorption and emission spectra intensities in the UV-VIS-NIR range have been found to depend on the polarity existing inside the pores of the network; in turn, this polarization can be tuned through the attachment of organic substituents to the tetrapyrrrole macrocycles before bonding them to the pore network. -
(DMSO)2Fe(II)TPP, (TMSO)2Fe(II)TPP, and (PSO)2Fe(II)TPP to Form a Transient Five-Coordinate Complex As Studied Using Transient Resonance Raman Spectroscopy
A Thesis entitled Photodissociation of (DMSO)2Fe(II)TPP, (TMSO)2Fe(II)TPP, and (PSO)2Fe(II)TPP to form a transient Five-Coordinate Complex as Studied Using Transient Resonance Raman Spectroscopy by Kenneth P. Boone Submitted in partial fulfillment of the requirements for the Master of Science Degree in Chemistry ___________________________________ Adviser: Dr. Eric W. Findsen ___________________________________ College of Graduate Studies University of Toledo December 2008 An Abstract of Photodissociation of (DMSO)2Fe(II)TPP, (TMSO)2Fe(II)TPP, and (PSO)2Fe(II)TPP to form a transient Five-Coordinate Complex as Studied Using Transient Resonance Raman Spectroscopy Kenneth P. Boone Submitted in partial fulfillment of the requirements for the Master of Science Degree in Chemistry University of Toledo December 2008 This work presents the results of UV-Visible absorption and Resonance Raman spectroscopic studies of three different heme complexes. (DMSO)2Fe(II)TPP (1) and (TMSO)2Fe(II)TPP (2) were studied in neat DMSO (dimethylsulfoxide) and neat TMSO (tetramethylene sulfoxide), respectively. A third heme complex, (PSO)2Fe(II)TPP (3), required a binary solvent of benzene and PSO (phenyl sulfoxide) in order to be created and studied. Both (1) and (2) were studied in the same binary solvent as well. The investigation determined that all three complexes behave similarly in relation to their UV-VIS and resonance Raman spectra. Resonance Raman experiments at low incident laser power have shown that (1), (2), and (3) exhibit the properties of a 6-coordinate, low- spin complex in the binary solvent. (1) and (2) exhibit the same properties in neat ii solvent. -
Matériaux Moléculaires Magnétiques À Base De Porphyrines Emel Önal
Matériaux moléculaires magnétiques à base de porphyrines Emel Önal To cite this version: Emel Önal. Matériaux moléculaires magnétiques à base de porphyrines. Material chemistry. Univer- sité Claude Bernard - Lyon I, 2014. English. NNT : 2014LYO10103. tel-01214515 HAL Id: tel-01214515 https://tel.archives-ouvertes.fr/tel-01214515 Submitted on 15 Oct 2015 HAL is a multi-disciplinary open access L’archive ouverte pluridisciplinaire HAL, est archive for the deposit and dissemination of sci- destinée au dépôt et à la diffusion de documents entific research documents, whether they are pub- scientifiques de niveau recherche, publiés ou non, lished or not. The documents may come from émanant des établissements d’enseignement et de teaching and research institutions in France or recherche français ou étrangers, des laboratoires abroad, or from public or private research centers. publics ou privés. 1XPHURG¶RUGUH $QQHH ŕʼnņŔņġŅņġō’ŖŏŊŗņœŔŊŕņġťŦġōŚŐŏġ ņŏġńŐŕŖŕņōōņġłŗņńġ ňņŃśņġŊŏŔŕŊŕŖŕņġŐŇġŕņńʼnŏŐōŐňŚġ ņńŐōņġŅŐńŕŐœłōņġŅņġńʼnŊŎŊņġ MOLECULAR MAGNETIC MATERIALS BASED ON PORPHYRIN MACROCYCLES VRXWHQXH SXEOLTXHPHQW OH -XLQ SDU 0(PHOgQDO JURY Mustafa Bulut Makoto Handa Catherine Hirel Dominique Luneau T.R. GEBZE INSTITUTE of TECHNOLOGY GRADUATE SCHOOL of ENGINEERING and SCIENCES MOLECULAR MAGNETIC MATERIALS BASED ON PORPHYRIN MACROCYCLES EMEL ÖNAL A THESIS SUBMITTED for THE DEGREE of DOCTOR of PHILOSOPHY CHEMISTRY DEPARTMENT GEBZE 2014 T.R. GEBZE INSTITUTE of TECHNOLOGY GRADUATE SCHOOL of ENGINEERING and SCIENCES MOLECULAR MAGNETIC MATERIALS BASED ON PORPHYRIN MACROCYCLES EMEL ÖNAL A THESIS SUBMITTED for THE DEGREE of DOCTOR of PHILOSOPHY CHEMISTRY DEPARTMENT THESIS SUPERVISOR ASST. PROF. DR. CATHERINE HIREL GEBZE 2014 SUMMARY The preparation of Molecule-Based Magnets is based on the assembling carriers of magnetic moment. These may be the metal ions only with diamagnetic linkers or the metal ions connected through open-shell organic molecule. -
Tetraphenylporphyrin Dimers
Tetraphenylporphyrin dimers An optical and magnetic resonance study CENTRALE I 4360 Promotor: dr. T. J. Schaafsma hoogleraar in de moleculaire fysica ^mzo\ X^ L. Benthem Tetraphenylporphyrin dimers An optical and magnetic resonance study Proefschrift ter verkrijging van de graad van doctor in de landbouwwetenschappen, , .£. BIBLIOTHEEK op gezag van de rector magnmcus, nKR dr. C. C. Oosterlee, L' ™ ^HOGESCHOOL in het openbaar te verdedigen op vrijdag 12 oktober 1984 des namiddags te vier uur in de aula van de Landbouwhogeschool te Wageningen \sW- \^£33-0,3 The author permitted to see the grand Academy of Lagado.Th e Academy largely described. The firstma n Isa wwa s ofmeagr e aspect,wit h sooty hands and face,hi s hair and beard long, ragged and singed in several places. His clothes, shirt, and skinwer e all of the same colour. He had been eight years upon aprojec t for extracting sunbeams out of cucumbers, whichwer e tob e put into vials hermetic ally sealed, and let out towar m the air in raw inclement summers. He toldme , he did not doubt in eightyear s more, that he should be able to supply the Governor's gardens with sunshine at a reasonable rate; but he complained that his stockwa s low,an d entreated me to give him something as an encouragement to ingenuity, especially since this had been aver y dear season for cucumbers. Jonathan Swift in GULLIVER'S TRAVELS. Eigenlijk zijn er maar twee dingen waar Foefje nooit aan zalwennen - en dat zijn tweelingen. De Bescheurkalender VanKoote n &D e Bie Vrijdag 12oktobe r 198A tériulloK ^% STELLINGEN. -
Photosensitized Singlet Oxygen and Its Applications
Coordination Chemistry Reviews 233Á/234 (2002) 351Á/371 www.elsevier.com/locate/ccr Photosensitized singlet oxygen and its applications Maria C. DeRosa, Robert J. Crutchley * Department of Chemistry, Ottawa-Carleton Chemistry Institute, Carleton University, 2240 Herzberg Building, 1125 Colonel By Drive, Ottawa, ON, Canada K1S 5B6 Received 18 September 2001; accepted 15 February 2002 Contents Abstract . ....................................................................... 351 1. Introduction ................................................................... 352 2. Properties of singlet oxygen ........................................................... 352 2.1 Electronic structure of singlet oxygen .................................................. 352 1 2.2 Quenching of O2 ............................................................. 352 2.3 Generation of singlet oxygen . ..................................................... 353 3. Types of photosensitizers ............................................................ 354 3.1 Organic dyes and aromatic hydrocarbons ............................................... 355 3.2 Porphyrins, phthalocyanines, and related tetrapyrroles ........................................ 355 3.3 Transition metal complexes . ..................................................... 357 3.4 Photobleaching of organic and metal complex photosensitizers ................................... 359 3.5 Semiconductors .............................................................. 359 3.6 Immobilized photosensitizers . .................................................... -
TETRAPHENYLPORPHYRIN DIMERS and THEIR DERIVATIVES by JESMAEL P. ^ZINGONI B.Sc, University of Zambia, 1975 a THESIS SUBMITTED IN
TETRAPHENYLPORPHYRIN DIMERS AND THEIR DERIVATIVES by JESMAEL P. ^ZINGONI B.Sc, University of Zambia, 1975 A THESIS SUBMITTED IN PARTIAL FULFILMENT OF THE REQUIREMENTS FOR THE DEGREE OF MASTER OF SCIENCE in THE FACULTY OF GRADUATE STUDIES in the Department of Chemistry We accept this thesis as conforming to the required standard THE UNIVERSITY OF BRITISH COLUMBIA May, 1979 Ci^Jesmael P. Zingoni In presenting this thesis in partial fulfilment of the requirements for an advanced degree at the University of British Columbia, I agree that the Library shall make it freely available for reference and study. I further agree that permission for extensive copying of this thesis for scholarly purposes may be granted by the Head of my Department or by his representatives. It is understood that copying or publication of this thesis for financial gain shall not be allowed without my written permission. Department of , CMSTRY The University of British Columbia 2075 Wesbrook Place Vancouver, Canada V6T 1W5 iw is, 1979 (ii) ABSTRACT Tetraphenylporphine (TPP) and its para-methyl deri• vative have been synthesized by direct reaction of pyrrole with the corresponding aldehyde. The synthesis of two unsymmetrically substituted tetraarylporphyrins is re• ported. The compounds prepared are' 5-(^-hydroxypheny1) -10,15j20-tritolyIporphyrin and 5-(^-hydroxypheny1)-10, 15}20-triphenyIporphyrin. The sy thesis of covalently linked porphyrin dimers, joined via ether linkages, is described. High yields of the tetraaryIporphyrin dimers were obtained by the reac• tion of the bi-functionall,6-ditosyloxyhexane with phenolic porphyrins such as 5-(^-hydroxypheny1)-10,15,20-tritoly1-" porphyrin. The dimeric porphyrins were then chromatograph- ically separated from the unreacted monomericLporphyrins , The reduction of the porphyrins(monomers and dimers) has been carried out using the standard diimide precursor, p-toluenesulfonylhydrazine. -
ACS Style Guide
➤ ➤ ➤ ➤ ➤ The ACS Style Guide ➤ ➤ ➤ ➤ ➤ THIRD EDITION The ACS Style Guide Effective Communication of Scientific Information Anne M. Coghill Lorrin R. Garson Editors AMERICAN CHEMICAL SOCIETY Washington, DC OXFORD UNIVERSITY PRESS New York Oxford 2006 Oxford University Press Oxford New York Athens Auckland Bangkok Bogotá Buenos Aires Calcutta Cape Town Chennai Dar es Salaam Delhi Florence Hong Kong Istanbul Karachi Kuala Lumpur Madrid Melbourne Mexico City Mumbai Nairobi Paris São Paulo Singapore Taipei Tokyo Toronto Warsaw and associated companies in Berlin Idaban Copyright © 2006 by the American Chemical Society, Washington, DC Developed and distributed in partnership by the American Chemical Society and Oxford University Press Published by Oxford University Press, Inc. 198 Madison Avenue, New York, NY 10016 Oxford is a registered trademark of Oxford University Press All rights reserved. No part of this publication may be reproduced, stored in a retrieval system, or transmitted, in any form or by any means, electronic, mechanical, photocopying, recording, or otherwise, without the prior permission of the American Chemical Society. Library of Congress Cataloging-in-Publication Data The ACS style guide : effective communication of scientific information.—3rd ed. / Anne M. Coghill [and] Lorrin R. Garson, editors. p. cm. Includes bibliographical references and index. ISBN-13: 978-0-8412-3999-9 (cloth : alk. paper) 1. Chemical literature—Authorship—Handbooks, manuals, etc. 2. Scientific literature— Authorship—Handbooks, manuals, etc. 3. English language—Style—Handbooks, manuals, etc. 4. Authorship—Style manuals. I. Coghill, Anne M. II. Garson, Lorrin R. III. American Chemical Society QD8.5.A25 2006 808'.06654—dc22 2006040668 1 3 5 7 9 8 6 4 2 Printed in the United States of America on acid-free paper ➤ ➤ ➤ ➤ ➤ Contents Foreword. -
Electrogenerated Chemiluminescence of Transition Metal Octaethylporphyrin and Tetraphenylporphyrin/Tri-N-Propylamine System
BearWorks MSU Graduate Theses Summer 2018 Electrogenerated Chemiluminescence of Transition Metal Octaethylporphyrin and Tetraphenylporphyrin/Tri-n-Propylamine System Jamie Lee Gray Missouri State University, [email protected] As with any intellectual project, the content and views expressed in this thesis may be considered objectionable by some readers. However, this student-scholar’s work has been judged to have academic value by the student’s thesis committee members trained in the discipline. The content and views expressed in this thesis are those of the student-scholar and are not endorsed by Missouri State University, its Graduate College, or its employees. Follow this and additional works at: https://bearworks.missouristate.edu/theses Part of the Chemicals and Drugs Commons, and the Chemistry Commons Recommended Citation Gray, Jamie Lee, "Electrogenerated Chemiluminescence of Transition Metal Octaethylporphyrin and Tetraphenylporphyrin/Tri-n-Propylamine System" (2018). MSU Graduate Theses. 3280. https://bearworks.missouristate.edu/theses/3280 This article or document was made available through BearWorks, the institutional repository of Missouri State University. The work contained in it may be protected by copyright and require permission of the copyright holder for reuse or redistribution. For more information, please contact [email protected]. ELECTROGENERATED CHEMILUMINESCENCE OF TRANSITION METAL OCTAETHYLPORPHYRIN AND TETRAPHENYLPORPHYRIN/TRI-N- PROPYLAMINE SYSTEMS A Masters Thesis Presented -
Local Optoelectronic Properties of Zinc-Porphyrin/Gold Molecular Interfaces
University of Pennsylvania ScholarlyCommons Publicly Accessible Penn Dissertations 2014 Local Optoelectronic Properties of Zinc-Porphyrin/Gold Molecular interfaces Xi Chen University of Pennsylvania, [email protected] Follow this and additional works at: https://repository.upenn.edu/edissertations Part of the Atomic, Molecular and Optical Physics Commons, and the Nanoscience and Nanotechnology Commons Recommended Citation Chen, Xi, "Local Optoelectronic Properties of Zinc-Porphyrin/Gold Molecular interfaces" (2014). Publicly Accessible Penn Dissertations. 1235. https://repository.upenn.edu/edissertations/1235 This paper is posted at ScholarlyCommons. https://repository.upenn.edu/edissertations/1235 For more information, please contact [email protected]. Local Optoelectronic Properties of Zinc-Porphyrin/Gold Molecular interfaces Abstract This research consists in designing a series of experiments to determine the molecular orbital energy levels of zinc-porphyrin molecule when vertically attached to Au(111) substrate. To study the zinc- porphyrine molecular orbitals we use visible light of different wavelengths. Thiolated zinc-porphyrin oligomer molecules link to Au(111) surface, embedded within an 1-octanethiol self-assembled monolayer. Current-Voltage characterization technique allow us to determine the electronic orbital structures of different zinc-porphyrin oligomer single molecules via scanning tunneling microscope. Coupling lasers of different wavelengths and the tunneling junction, illumination effect on the molecular orbital energy levels of zinc-porphyrin molecules is investigated. The results indicate that the experimental zinc-porphyrin orbital energy levels are qualitatively consistent with previous calculations and experiments of similar porphyrin molecules. With illumination at given wavelengths, HOMO-LUMO gaps decreases for zinc- porphyrin molecules, and under dark condition the dimer zinc-porphyrin molecule shows a larger HOMO- LUMO gap than the monomer counterpart.