Molecular Characterization of Spinach (Spinacia
Total Page:16
File Type:pdf, Size:1020Kb
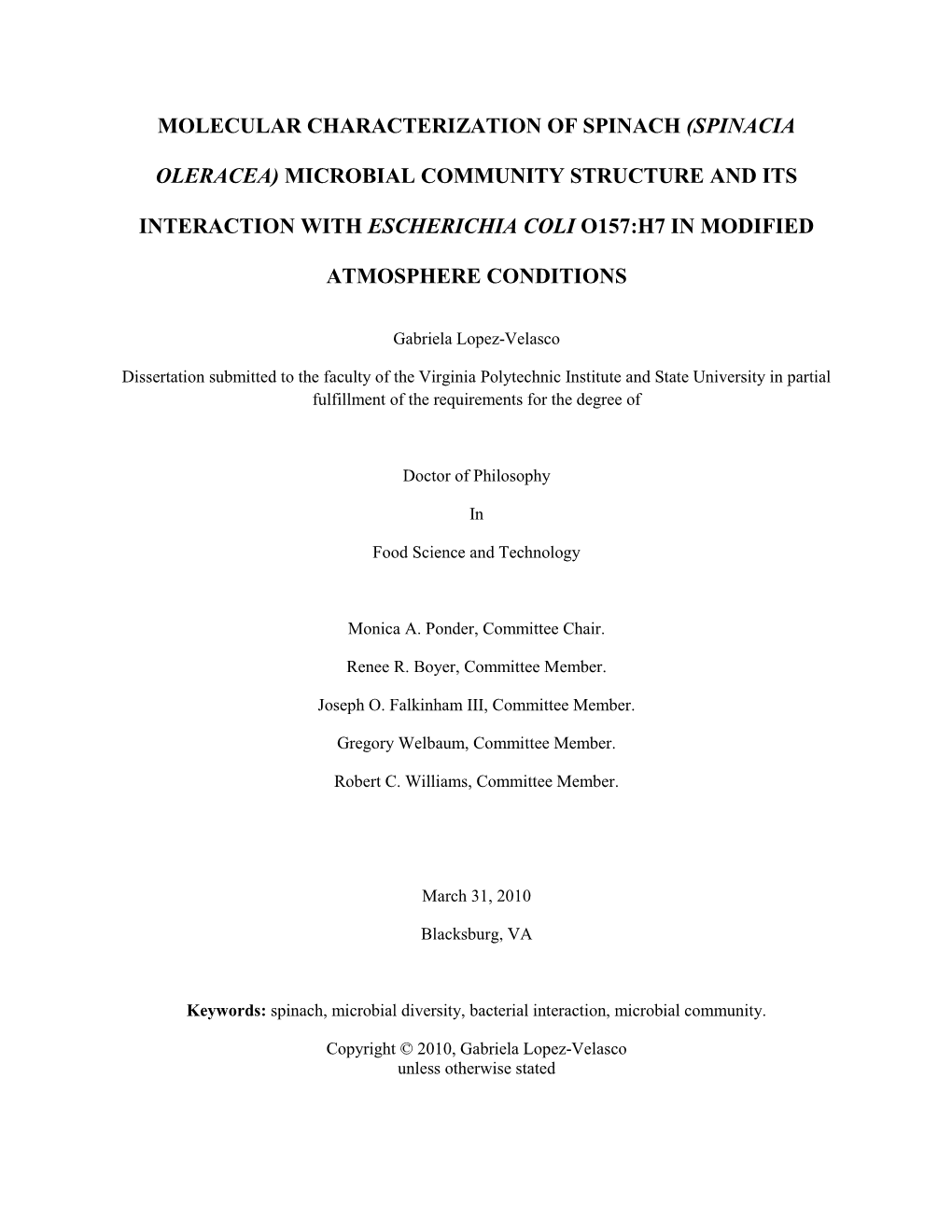
Load more
Recommended publications
-
The Vascular Plants of Massachusetts
The Vascular Plants of Massachusetts: The Vascular Plants of Massachusetts: A County Checklist • First Revision Melissa Dow Cullina, Bryan Connolly, Bruce Sorrie and Paul Somers Somers Bruce Sorrie and Paul Connolly, Bryan Cullina, Melissa Dow Revision • First A County Checklist Plants of Massachusetts: Vascular The A County Checklist First Revision Melissa Dow Cullina, Bryan Connolly, Bruce Sorrie and Paul Somers Massachusetts Natural Heritage & Endangered Species Program Massachusetts Division of Fisheries and Wildlife Natural Heritage & Endangered Species Program The Natural Heritage & Endangered Species Program (NHESP), part of the Massachusetts Division of Fisheries and Wildlife, is one of the programs forming the Natural Heritage network. NHESP is responsible for the conservation and protection of hundreds of species that are not hunted, fished, trapped, or commercially harvested in the state. The Program's highest priority is protecting the 176 species of vertebrate and invertebrate animals and 259 species of native plants that are officially listed as Endangered, Threatened or of Special Concern in Massachusetts. Endangered species conservation in Massachusetts depends on you! A major source of funding for the protection of rare and endangered species comes from voluntary donations on state income tax forms. Contributions go to the Natural Heritage & Endangered Species Fund, which provides a portion of the operating budget for the Natural Heritage & Endangered Species Program. NHESP protects rare species through biological inventory, -
'USDA Red' Spinach
HORTSCIENCE 54(11):2070–2072. 2019. https://doi.org/10.21273/HORTSCI14308-19 cultivars. It has a monoecious flowering habit and produces smooth seeds. Compared with other varieties, it has moderate resistance to ‘USDA Red’ Spinach bolting. Beiquan Mou Field evaluations. ‘USDA Red’ was planted in a field at the experiment station U.S. Department of Agriculture (USDA), Agricultural Research Service, of the USDA in Aug. 2015, Aug. 2016, Aug. 1636 East Alisal Street, Salinas, CA 93905 2017, and Aug. 2018 in Salinas, CA, to Additional index words. antioxidant capacity, betacyanin, nutritional value, red leaf, Spinacia evaluate its horticultural and nutritional traits. A green-leaf spinach cultivar, Polar oleracea Bear (Rijk Zwaan, De Lier, Holland), and two red-veined cultivars, Bordeaux and Red Spinach has always been known as a green that attack proteins, lipids, and DNA, conse- Deer (Rijk Zwaan), were included in the leafy vegetable. Although there are some quently leading to damage and dysfunction of trials. The experiment design was a random- plants called ‘‘red spinach,’’ they are usually enzymes, cell membranes, and genetic mate- ized complete block with four replications. red-leaf amaranth (Amaranthus spp.) or other rial (Stintzing and Carle, 2004). Betacyanin Each plot consisted of 50 plants of a geno- species (e.g., Blitum rubrum), not true spinach has been shown to significantly reduce oxi- type, with 30 cm between plants and 35 cm (Spinacia oleracea). There are currently some dative stress in patients and may help in between rows on 1-m wide double-row beds. ‘‘red’’ true spinach cultivars on the market, but preventing chronic pathologies, inflamma- Ten plants were randomly selected from each the red coloration is limited to the veins of the tion, and cancer (Stintzing and Carle, 2004; plot to measure petiole length, spread (di- leaves. -
Chenopodioideae, Chenopodiaceae/ Amaranthaceae): Implications for Evolution and Taxonomy
Fruit and Seed Anatomy of Chenopodium and Related Genera (Chenopodioideae, Chenopodiaceae/ Amaranthaceae): Implications for Evolution and Taxonomy Alexander P. Sukhorukov1,2*, Mingli Zhang1,3 1 Key Laboratory of Biogeography and Bioresource in Arid Land, Xinjiang Institute of Ecology and Geography, Chinese Academy of Sciences, Urumqi, Xinjiang, China, 2 Department of Higher Plants, Biological Faculty, Moscow Lomonosov State University, Moscow, Russia, 3 Institute of Botany, Chinese Academy of Sciences, Beijing, China Abstract A comparative carpological study of 96 species of all clades formerly considered as the tribe Chenopodieae has been conducted for the first time. The results show important differences in the anatomical structure of the pericarp and seed coat between representatives of terminal clades including Chenopodium s.str.+Chenopodiastrum and the recently recognized genera Blitum, Oxybasis and Dysphania. Within Chenopodium the most significant changes in fruit and seed structure are found in members of C. sect. Skottsbergia. The genera Rhagodia and Einadia differ insignificantly from Chenopodium. The evolution of heterospermy in Chenopodium is discussed. Almost all representatives of the tribe Dysphanieae are clearly separated from other Chenopodioideae on the basis of a diverse set of characteristics, including the small dimensions of the fruits (especially in Australian taxa), their subglobose shape (excl. Teloxys and Suckleya), and peculiarities of the pericarp indumentum. The set of fruit and seed characters evolved within the subfamily Chenopodioideae is described. A recent phylogenetic hypothesis is employed to examine the evolution of three (out of a total of 21) characters, namely seed color, testa-cell protoplast characteristics and embryo orientation. Citation: Sukhorukov AP, Zhang M (2013) Fruit and Seed Anatomy of Chenopodium and Related Genera (Chenopodioideae, Chenopodiaceae/Amaranthaceae): Implications for Evolution and Taxonomy. -
Phylogeny and Comparative Analysis of Chinese Chamaesium Species Revealed by the Complete Plastid Genome
plants Article Phylogeny and Comparative Analysis of Chinese Chamaesium Species Revealed by the Complete Plastid Genome 1 1, 2 1, 1, Xian-Lin Guo , Hong-Yi Zheng y, Megan Price , Song-Dong Zhou * and Xing-Jin He * 1 Key Laboratory of Bio-Resources and Eco-Environment of Ministry of Education, College of Life Sciences, Sichuan University, Chengdu 610065, China; [email protected] (X.-L.G.); [email protected] (H.-Y.Z.) 2 Sichuan Key Laboratory of Conservation Biology on Endangered Wildlife, College of Life Sciences, Sichuan University, Chengdu 610065, China; [email protected] * Correspondence: [email protected] (S.-D.Z.); [email protected] (X.-J.H.) Equal contributions to this work. y Received: 30 June 2020; Accepted: 28 July 2020; Published: 30 July 2020 Abstract: Chamaesium H. Wolff (Apiaceae, Apioideae) is a small genus mainly distributed in the Hengduan Mountains and the Himalayas. Ten species of Chamaesium have been described and nine species are distributed in China. Recent advances in molecular phylogenetics have revolutionized our understanding of Chinese Chamaesium taxonomy and evolution. However, an accurate phylogenetic relationship in Chamaesium based on the second-generation sequencing technology remains poorly understood. Here, we newly assembled nine plastid genomes from the nine Chinese Chamaesium species and combined these genomes with eight other species from five genera to perform a phylogenic analysis by maximum likelihood (ML) using the complete plastid genome and analyzed genome structure, GC content, species pairwise Ka/Ks ratios and the simple sequence repeat (SSR) component. We found that the nine species’ plastid genomes ranged from 152,703 bp (C. -
Draft Genome of Spinach and Transcriptome Diversity of 120 Spinacia Accessions
ARTICLE Received 20 Jun 2016 | Accepted 7 Mar 2017 | Published 24 May 2017 DOI: 10.1038/ncomms15275 OPEN Draft genome of spinach and transcriptome diversity of 120 Spinacia accessions Chenxi Xu1,*, Chen Jiao2,*, Honghe Sun2,*, Xiaofeng Cai1, Xiaoli Wang1, Chenhui Ge1, Yi Zheng2, Wenli Liu2, Xuepeng Sun2, Yimin Xu2, Jie Deng3, Zhonghua Zhang3, Sanwen Huang3, Shaojun Dai1, Beiquan Mou4, Quanxi Wang1, Zhangjun Fei1,2,5 & Quanhua Wang1 Spinach is an important leafy vegetable enriched with multiple necessary nutrients. Here we report the draft genome sequence of spinach (Spinacia oleracea,2n¼ 12), which contains 25,495 protein-coding genes. The spinach genome is highly repetitive with 74.4% of its content in the form of transposable elements. No recent whole genome duplication events are observed in spinach. Genome syntenic analysis between spinach and sugar beet suggests substantial inter- and intra-chromosome rearrangements during the Caryophyllales genome evolution. Transcriptome sequencing of 120 cultivated and wild spinach accessions reveals more than 420 K variants. Our data suggests that S. turkestanica is likely the direct progenitor of cultivated spinach and spinach domestication has a weak bottleneck. We identify 93 domestication sweeps in the spinach genome, some of which are associated with important agronomic traits including bolting, flowering and leaf numbers. This study offers insights into spinach evolution and domestication and provides resources for spinach research and improvement. 1 Development and Collaborative Innovation Center of Plant Germplasm Resources, College of Life and Environmental Sciences, Shanghai Normal University, Shanghai 200234, China. 2 Boyce Thompson Institute, Cornell University, Ithaca, New York 14853, USA. 3 Institute of Vegetables and Flowers, Chinese Academy of Agricultural Sciences, Beijing 100081, China. -
An Illustrated Key to the Amaranthaceae of Alberta
AN ILLUSTRATED KEY TO THE AMARANTHACEAE OF ALBERTA Compiled and writen by Lorna Allen & Linda Kershaw April 2019 © Linda J. Kershaw & Lorna Allen This key was compiled using informaton primarily from Moss (1983), Douglas et. al. (1998a [Amaranthaceae], 1998b [Chenopodiaceae]) and the Flora North America Associaton (2008). Taxonomy follows VASCAN (Brouillet, 2015). Please let us know if there are ways in which the key can be improved. The 2015 S-ranks of rare species (S1; S1S2; S2; S2S3; SU, according to ACIMS, 2015) are noted in superscript (S1;S2;SU) afer the species names. For more details go to the ACIMS web site. Similarly, exotc species are followed by a superscript X, XX if noxious and XXX if prohibited noxious (X; XX; XXX) according to the Alberta Weed Control Act (2016). AMARANTHACEAE Amaranth Family [includes Chenopodiaceae] Key to Genera 01a Flowers with spiny, dry, thin and translucent 1a (not green) bracts at the base; tepals dry, thin and translucent; separate ♂ and ♀ fowers on same the plant; annual herbs; fruits thin-walled (utricles), splitting open around the middle 2a (circumscissile) .............Amaranthus 01b Flowers without spiny, dry, thin, translucent bracts; tepals herbaceous or feshy, greenish; fowers various; annual or perennial, herbs or shrubs; fruits various, not splitting open around the middle ..........................02 02a Leaves scale-like, paired (opposite); stems feshy/succulent, with fowers sunk into stem; plants of saline habitats ... Salicornia rubra 3a ................. [Salicornia europaea] 02b Leaves well developed, not scale-like; stems not feshy; plants of various habitats. .03 03a Flower bracts tipped with spine or spine-like bristle; leaves spine-tipped, linear to awl- 5a shaped, usually not feshy; tepals winged from the lower surface .............. -
Towards a Species Level Tree of the Globally Diverse Genus
Molecular Phylogenetics and Evolution 62 (2012) 359–374 Contents lists available at SciVerse ScienceDirect Molecular Phylogenetics and Evolution journal homepage: www.elsevier.com/locate/ympev Towards a species level tree of the globally diverse genus Chenopodium (Chenopodiaceae) ⇑ Susy Fuentes-Bazan a,b, Guilhem Mansion a, Thomas Borsch a, a Botanischer Garten und Botanisches Museum Berlin-Dahlem und Institut für Biologie, Freie Universität Berlin, Dahlem Centre of Plant Sciences, Königin-Luise-Straße 6-8, 14195 Berlin, Germany b Herbario Nacional de Bolivia, Universidad Mayor de San Andrés (UMSA), La Paz, Bolivia article info abstract Article history: Chenopodium is a large and morphologically variable genus of annual and perennial herbs with an almost Received 21 March 2011 global distribution. All subgenera and most sections of Chenopodium were sampled along with other gen- Revised 28 September 2011 era of Chenopodieae, Atripliceae and Axyrideae across the subfamily Chenopodioideae (Chenopodiaceae), Accepted 11 October 2011 totalling to 140 taxa. Using Maximum parsimony and Bayesian analyses of the non-coding trnL-F Available online 24 October 2011 (cpDNA) and nuclear ITS regions, we provide a comprehensive picture of relationships of Chenopodium sensu lato. The genus as broadly classified is highly paraphyletic within Chenopodioideae, consisting of Keywords: five major clades. Compared to previous studies, the tribe Dysphanieae with three genera Dysphania, Tel- Chenopodium oxys and Suckleya (comprising the aromatic species of Chenopodium s.l.) is now shown to form one of the Chenopodioideae Chenopodieae early branches in the tree of Chenopodioideae. We further recognize the tribe Spinacieae to include Spina- TrnL-F cia, several species of Chenopodium, and the genera Monolepis and Scleroblitum. -
FLORA of BEIJING Jinshuang Ma and Quanru Liu
URBAN HABITATS, VOLUME 1, NUMBER 1 • ISSN 1541-7115 FLORA OF BEIJING http://www.urbanhabitats.org Jinshuang Ma and Quanru Liu Flora of Beijing: An Overview and Suggestions for Future Research* Jinshuang Ma and Quanru Liu Brooklyn Botanic Garden, 1000 Washington Avenue, Brooklyn, New York 11225; [email protected]; [email protected] nonnative, invasive, and weed species, as well as a lst Abstract This paper reviews Flora of Beijing (He, 1992), of relevant herbarium collections. We also make especially from the perspective of the standards of suggestions for future revisions of Flora of Beijing in modern urban floras of western countries. The the areas of description and taxonomy. We geography, land-use and population patterns, and recommend more detailed categorization of species vegetation of Beijing are discussed, as well as the by origin (from native to cultivated, including plants history of Flora of Beijing. The vegetation of Beijing, introduced, escaped, and naturalized from gardens which is situated in northern China, has been and parks); by scale and scope of distribution drastically altered by human activities; as a result, it (detailing from worldwide to special or unique local is no longer characterized by the pine-oak mixed distribution); by conservation ranking (using IUCN broad-leaved deciduous forests typical of the standards, for example); by habitat; and by utilization. northern temperate region. Of the native species that Finally, regarding plant treatments, we suggest remain, the following dominate: Pinus tabuliformis, improvements in the stability of nomenclature, Quercus spp., Acer spp., Koelreuteria paniculata, descriptions of taxa, and the quality and quantity of Vitex negundo var. -
Scientific Classification
Entry prepared by Sarah Mortati „08 in College Seminar 235 Food for Thought: The Science, Culture, & Politics of Food Spring 2008 SPINACH SCIENTIFIC CLASSIFICATION AND ENTYMOLOGY Scientific Classification Spinach, or “roundleaf spinage”, is a staple of the Kingdom Plantae early American vegetable gardens. It is a Phylum Magnoliophyta relatively quick-growing vegetable and easy to Class Magnoliopsida maintain. Spinach is in the classification system Order Caryophyllales Family Amaranthaceae. spinacia oleraceae being its Family Amaranthaceae official scientific classification name. Within Genus Spinacia Amaranthaceae there are about 102 genera and 1400 Species s. oleraceae species world wide. It is within the family of leafy http://www.usda.gov/wps/portal/usdahome1 green vegetables, referred to as „greens‟ or „potherbs‟, because they were historically cooked before eating. Spinach ranges in color from light to dark green and comes in two general types: the crinkle leaf variety and the smooth leaf variety, although there are varieties that contain characters of both, known as “semi- Savoy Spinach”2 The word “spinach” is derived from the Persian word “ispanai” which means „green hand‟, which later became „spanachia‟ in late Latin, and ultimately „spinage‟ and then „spinach‟ in English.3 4 1 USDA, United States Department of Agriculture http://www.usda.gov/wps/portal/usdahome 2 Kenneth F. Kiple. Cambridge World History of Food. (United Kingdom: Cambridge, 2002) 1857 p 288 3 UGA Horticulture, Spinach University of Georgia College of Agricultural and Environmental Sciences, Department of Horticulture. Vegetable Crops http://www.uga.edu/vegetable/spinach.html 4 Flickr.com (Creative Commons) HISTORICAL IMPORTANCE Spinach is native to southwestern Asia. -
Phytochemical Investigations of Spinacia Oleracea : an Important Leafy Vegetable Used in Indian Diet
Available online a t www.scholarsresearchlibrary.com Scholars Research Library Central European Journal of Experimental Biology , 2015, 4 (1):1-4 (http://scholarsresearchlibrary.com/archive.html) ISSN: 2278–7364 Phytochemical Investigations of Spinacia oleracea : An important leafy vegetable used in Indian Diet Pramod C. Mane, Deepali D. Kadam, Ravindra D. Chaudhari *, Kanchan A. Varpe, Sunita D. Sarogade, Vrushali T. Thorat, Siddhi S. Said and Shabnam A. R. Sayyed Zoology Research Centre, Shri Shiv Chhatrapati College of Arts, Commerce and Science, Junnar, Dist. Pune (M.S.), Savitribai Phule Pune University, India. _____________________________________________________________________________________________ ABSTRACT The nutritional and medicinal benefits of vegetables provide a better support for human wellbeing. There are several edible vegetables which are used in day to day kitchen in different forms. The usages of green leafy vegetables are limited to a specific geographical location. In the present work, we have investigated phytochemicals of Spinacia oleracea, one of the most important vegetable used in India. The presence of phytochemicals including phytosterols, saponins, alkaloids, phenolic compounds and tannins, proteins, glycosides, flavonoids, carbohydrates, quinones, coumerin, terpenoids, anthocyanins and emodins were determined in the Spinacia oleracea. The extract of Spinacia oleracea exhibits the presence of phytosterols, proteins, glycosides, flavonoids, carbohydrates, quinones, and terpenoids. It was concluded that -
Influence of Row Spacing and Frequency of Cuttings on Spinach (<Em>Spinacia Oleracea</Em>) Production
Journal of Bioresource Management Volume 3 Issue 1 Article 9 Influence of Row Spacing and rF equency of Cuttings on Spinach (Spinacia oleracea) Production Daud Ahmad Awan CABI Central and West Asia, Rawalpindi, Pakistan Faheem Ahmad COMSATS Institute of Information Technology, Islamabad, Pakistan, [email protected] Madeeha Imdad CABI Central and West Asia, Rawalpindi, Pakistan Follow this and additional works at: https://corescholar.libraries.wright.edu/jbm Part of the Biodiversity Commons, and the Biology Commons Recommended Citation Awan, D. A., Ahmad, F., & Imdad, M. (2016). Influence of Row Spacing and rF equency of Cuttings on Spinach (Spinacia oleracea) Production, Journal of Bioresource Management, 3 (1). DOI: https://doi.org/10.35691/JBM.6102.0047 ISSN: 2309-3854 online This Article is brought to you for free and open access by CORE Scholar. It has been accepted for inclusion in Journal of Bioresource Management by an authorized editor of CORE Scholar. For more information, please contact [email protected]. Influence of Row Spacing and rF equency of Cuttings on Spinach (Spinacia oleracea) Production © Copyrights of all the papers published in Journal of Bioresource Management are with its publisher, Center for Bioresource Research (CBR) Islamabad, Pakistan. This permits anyone to copy, redistribute, remix, transmit and adapt the work for non-commercial purposes provided the original work and source is appropriately cited. Journal of Bioresource Management does not grant you any other rights in relation to this website or the material on this website. In other words, all other rights are reserved. For the avoidance of doubt, you must not adapt, edit, change, transform, publish, republish, distribute, redistribute, broadcast, rebroadcast or show or play in public this website or the material on this website (in any form or media) without appropriately and conspicuously citing the original work and source or Journal of Bioresource Management’s prior written permission. -
EPPO Reporting Service
ORGANISATION EUROPEENNE EUROPEAN AND MEDITERRANEAN ET MEDITERRANEENNE PLANT PROTECTION POUR LA PROTECTION DES PLANTES ORGANIZATION EPPO Reporting Service NO. 2 PARIS, 2019-02 General 2019/027 New data on quarantine pests and pests of the EPPO Alert List 2019/028 EPPO report on notifications of non-compliance Pests 2019/029 First report of Spodoptera frugiperda in China 2019/030 First finding of Euwallacea fornicatus in Poland 2019/031 First report of Halyomorpha halys in Turkey 2019/032 First report of Oligonychus perseae in Morocco 2019/033 First report of Penthimiola bella in Morocco 2019/034 Dead beetle of Popillia japonica found in trap at Schiphol airport (NL) 2019/035 Studies on the native range of Agrilus planipennis 2019/036 Studies on host plants of Scirtothrips dorsalis in the Palm House at Kew Gardens (United Kingdom) 2019/037 First report of Globodera pallida in Estonia 2019/038 First report of Meloidogyne fallax in Sweden 2019/039 First report of Achatina fulica in Italy Diseases 2019/040 Studies on pine needle blight pathogens in Europe and USA 2019/041 First report of Lecanosticta acicola in Sweden 2019/042 First report of Dothistroma pini in Germany Invasive plants 2019/043 First report of several new cactus species from Gran Canaria (ES) 2019/044 First report of Mahonia lomariifolia in South Africa 2019/045 First report of Euphorbia serpens and Euphorbia glyptosperma in Romania 2019/046 First report of the casual occurrence of Handroanthus heptaphyllus in Sicily (IT) 2019/047 Update on the exotic plant species of continental Portugal 2019/048 Joint ESENIAS and DIAS Scientific Conference and 9th ESENIAS Workshop (Republic of Macedonia, 2019-09—03/06) 21 Bld Richard Lenoir Tel: 33 1 45 20 77 94 Web: www.eppo.int 75011 Paris E-mail: [email protected] GD: gd.eppo.int EPPO Reporting Service 2019 no.