Mechanical Properties of Trabecular Meshwork
Total Page:16
File Type:pdf, Size:1020Kb
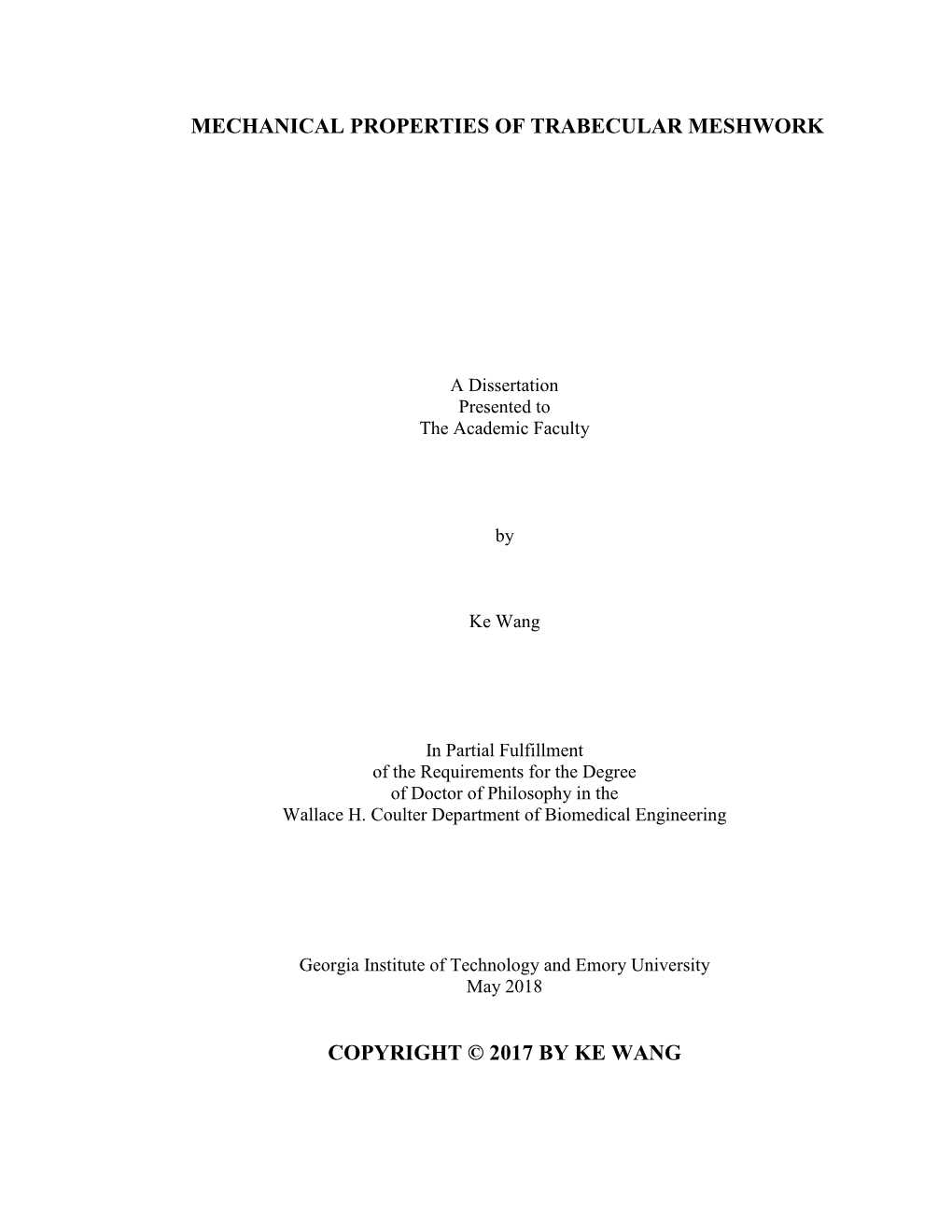
Load more
Recommended publications
-
Experimental Glaucoma Model with Controllable Intraocular Pressure History Kayla R
www.nature.com/scientificreports OPEN Experimental glaucoma model with controllable intraocular pressure history Kayla R. Ficarrotta1, Youssef H. Mohamed1 & Christopher L. Passaglia1,2* Glaucoma-like neuropathies can be experimentally induced by disturbing aqueous outfow from the eye, resulting in intraocular pressure (IOP) changes that are variable in magnitude and time course and permanent in duration. This study introduces a novel method of glaucoma induction that ofers researchers round-the-clock measurement and reversible control of IOP for the frst time. One eye of Brown-Norway rats was implanted with a cannula tethered to a pressure sensor and aqueous reservoir. IOP was raised 10 mmHg for weeks-to-months in treated animals and unaltered in control animals. Counts of Brn3a-expressing retinal ganglion cells (RGCs) in implanted eyes were indistinguishable from non-implanted eyes in control animals and 15 ± 2%, 23 ± 4%, and 38 ± 4% lower in animals exposed to 2, 4, and 9 weeks of IOP elevation. RGC loss was greater in peripheral retina at 2 weeks and widespread at longer durations. Optic nerves also showed progressive degeneration with exposure duration, yet conventional outfow facility of implanted eyes was normal (24.1 ± 2.9 nl/min/mmHg) even after 9-weeks elevation. Hence, this infusion-based glaucoma model exhibits graded neural damage with unimpaired outfow pathways. The model further revealed a potentially-signifcant fnding that outfow properties of rat eyes do not remodel in response to chronic ocular hypertension. Glaucoma is a heterogeneous group of ocular disorders characterized by progressive and preferential loss of ret- inal ganglion cells (RGCs), resulting in visual feld defcits and ultimately blindness. -
Japanese Journal of Ophthalmology Vol.43 No.4
Autonomic Nerves Containing Substance P in the Aqueous Outflow Channels and Scleral Spur of the Guinea Pig Mariko Sasamoto, Hai-Bo Chen and Shigeo Tsukahara Department of Ophthalmology, Yamanashi Medical University, Tamaho, Yamanashi, Japan Purpose: To study the innervation of the aqueous outflow channels and scleral spur by auto- nomic nerves containing substance P. Methods: The experiments were conducted on guinea pigs. Immunohistochemical tech- niques and capsaicin-ablation of the sensory nerves were used to investigate nerves contain- ing substance P at the light and electron microscopic level. Results: Nerves containing substance P were observed in the aqueous outflow channels and scleral spur regions. The fine structures of these nerves had a similar pattern in those regions, and the labeled elements had abundant small vesicles, a few large vesicles, and numerous neurotubuli. Following capsaicin treatment, these nerves remained intact and no degener- ated substance P-like immunoreactive nerves were found. Conclusions: Nerves containing substance P are most likely of autonomic origin in view of their ultrastructural features. These nerves innervate the aqueous outflow channels and scleral spur, and are probably important for neurogenic influences on the intraocular pres- sure by the autonomic nervous system. Jpn J Ophthalmol 1999;43:272–278 © 1999 Japa- nese Ophthalmological Society Key Words: Aqueous outflow channels, capsaicin, guinea pig, immunohistochemistry, substance P. Introduction The scleral spur region should also be considered 4,5 Aqueous outflow channels and the scleral spur because it plays a part in outflow regulation. The human scleral spur contains elastic tissue, similar to play important roles in the regulation of intraocular sclera, so that changes in the IOP might affect these pressure (IOP), and are known to have varied pepti- 5 dergic innervation, which have received special at- tissues. -
Tissue-Engineered Models for Glaucoma Research
micromachines Review Tissue-Engineered Models for Glaucoma Research Renhao Lu 1 , Paul A. Soden 2 and Esak Lee 1,* 1 Nancy E. and Peter C. Meinig School of Biomedical Engineering, Cornell University, Ithaca, NY 14853, USA; [email protected] 2 College of Human Ecology, Cornell University, Ithaca, NY 14853, USA; [email protected] * Correspondence: [email protected]; Tel.: +1-607-255-8491 Received: 5 June 2020; Accepted: 22 June 2020; Published: 24 June 2020 Abstract: Glaucoma is a group of optic neuropathies characterized by the progressive degeneration of retinal ganglion cells (RGCs). Patients with glaucoma generally experience elevations in intraocular pressure (IOP), followed by RGC death, peripheral vision loss and eventually blindness. However, despite the substantial economic and health-related impact of glaucoma-related morbidity worldwide, the surgical and pharmacological management of glaucoma is still limited to maintaining IOP within a normal range. This is in large part because the underlying molecular and biophysical mechanisms by which glaucomatous changes occur are still unclear. In the present review article, we describe current tissue-engineered models of the intraocular space that aim to advance the state of glaucoma research. Specifically, we critically evaluate and compare both 2D and 3D-culture models of the trabecular meshwork and nerve fiber layer, both of which are key players in glaucoma pathophysiology. Finally, we point out the need for novel organ-on-a-chip models of glaucoma that functionally integrate currently available 3D models of the retina and the trabecular outflow pathway. Keywords: glaucoma; tissue engineering; trabecular meshwork; Schlemm’s canal; retinal ganglion cell; intraocular pressure; optic nerve head; electrospinning; soft lithography; 3D scaffold; 3D bioprinting 1. -
Nomina Histologica Veterinaria, First Edition
NOMINA HISTOLOGICA VETERINARIA Submitted by the International Committee on Veterinary Histological Nomenclature (ICVHN) to the World Association of Veterinary Anatomists Published on the website of the World Association of Veterinary Anatomists www.wava-amav.org 2017 CONTENTS Introduction i Principles of term construction in N.H.V. iii Cytologia – Cytology 1 Textus epithelialis – Epithelial tissue 10 Textus connectivus – Connective tissue 13 Sanguis et Lympha – Blood and Lymph 17 Textus muscularis – Muscle tissue 19 Textus nervosus – Nerve tissue 20 Splanchnologia – Viscera 23 Systema digestorium – Digestive system 24 Systema respiratorium – Respiratory system 32 Systema urinarium – Urinary system 35 Organa genitalia masculina – Male genital system 38 Organa genitalia feminina – Female genital system 42 Systema endocrinum – Endocrine system 45 Systema cardiovasculare et lymphaticum [Angiologia] – Cardiovascular and lymphatic system 47 Systema nervosum – Nervous system 52 Receptores sensorii et Organa sensuum – Sensory receptors and Sense organs 58 Integumentum – Integument 64 INTRODUCTION The preparations leading to the publication of the present first edition of the Nomina Histologica Veterinaria has a long history spanning more than 50 years. Under the auspices of the World Association of Veterinary Anatomists (W.A.V.A.), the International Committee on Veterinary Anatomical Nomenclature (I.C.V.A.N.) appointed in Giessen, 1965, a Subcommittee on Histology and Embryology which started a working relation with the Subcommittee on Histology of the former International Anatomical Nomenclature Committee. In Mexico City, 1971, this Subcommittee presented a document entitled Nomina Histologica Veterinaria: A Working Draft as a basis for the continued work of the newly-appointed Subcommittee on Histological Nomenclature. This resulted in the editing of the Nomina Histologica Veterinaria: A Working Draft II (Toulouse, 1974), followed by preparations for publication of a Nomina Histologica Veterinaria. -
The Narrow Range of Intraocular Pressure (TOP) (12-20Mmhg)
J. Smooth Muscle Res. 32: 229•`247, 1996. Review Ocular Outflow Facility with Emphasis on Neuronal Regulation of Intraocular Smooth Muscles Ryo SUZUKI, MD Department of Ophthalmology, Yamaguchi University School of Medicine, Ube City, 755, Japan The narrow range of intraocular pressure (TOP) (12-20mmHg) in normal individuals has stimulated a search for possible regulatory mechanisms7,18,65) of aqueous production and outflow6,46). Compared with aqueous production, the aqueous outflow mechanisms of the neuronal, humoral, and mechanical processes have been studied much less. Because the eye constitutes a small portion of total body mass, it is very difficult to determine the neuronal and mechanical regulations of intraocular muscle and outflow facility. Locally acting mechanisms, should be an ideal means for integrating its physiology58,76). The peripheral nervous system is designed to effect such local control. Because most available antiglaucoma agents interact with the autonomic mechanisms and mechanical activities of the smooth muscles in the eye53,59,68),combined studies of the intraocular muscles with eye perfusion7,66) and cell shape changes of cultured cells from the outflow route22,66) would suggest the role of the nervous system in regulating IOP. From an historical view point, much speculation and minimal experimentation have been focused on the influence of the iris sphincter, the iris dilator, and the ciliary muscles on aqueous humor outflow. Accommodation, cholinergic agonists, and stimulation of the oculomotor nerve, all increase outflow facility19), whereas ganglionic blocking agents and anticholinergic drugs decrease the ocular outflow facility5,66). Furthermore, the outflow facility increase with intravenous pilocarpine administration is instantaneous, suggesting that the effect is mediated by an arterially perfused tissue31,32,46). -
Simultaneous Influence of Sympathetic Autonomic Stress on Schlemm's
www.nature.com/scientificreports OPEN Simultaneous infuence of sympathetic autonomic stress on Schlemm’s canal, intraocular pressure and ocular circulation Wei Chen, Zhiqi Chen, Yan Xiang, Chaohua Deng, Hong Zhang & Junming Wang* This study aimed to investigate changes in Schlemm’s canal, intraocular pressure and ocular blood circulation following the activation of the sympathetic nervous system. Twenty healthy volunteers were enrolled in this study. The cold pressor test (CPT) was adopted. Cross-sectional area of Schlemm’s canal (SCAR), superfcial and deep retinal vessel densities (s-RVD;d-RVD), pupil diameter (PD), intraocular pressure (IOP), mean ocular perfusion pressure (MOPP) and heart rate variability (HRV) were measured at three time-points: baseline (T0) and 5 min (T1) and 10 min (T2) after the CPT. After cold stimulation, LF/HF index (the ratio of low frenquency and high frenquency) increased signifcantly. IOP decreased from 16.9 ± 1.9 mmHg at baseline to 16.4 ± 2.7 mmHg at T1 and to 15.2 ± 2.7 mmHg at T2. The nasal cross-sectional area of SCAR (SCAR-n) increased from 6283.9 ± 2696.2 µm2 at baseline to 8392.9 ± 3258.7 µm2 at T1 and to 10422.0 ± 3643.8 µm2 at T2. The temporal cross-sectional area of SCAR (SCAR-t) increased from 6414.5 ± 2218.7 µm2 at baseline to 8610.8 ± 2317.1 µm2 at T1 and to 11544.0 ± 4129.2 µm2 at T2. The expansion of Schlemm’s canal was observed after the CPT might be caused by sympathetic nerve stimulation, subsequently leading to decreased IOP. -
Human Trabecular Mesh Work Organ Culture: Morphology and Glycosaminoglycan Synthesis
Investigative Ophthalmology & Visual Science, Vol. 29, No. 1, January 1988 Copyright © Association for Research in Vision and Ophthalmology Human Trabecular Mesh work Organ Culture: Morphology and Glycosaminoglycan Synthesis Ted 5. Acott,* Paul D. Kingsley, John R. Samples, and E. Michael Van Buskirk Human corneoscleral explants were maintained for several weeks in defined, serum-free media. Tra- becular cell vitality, as judged by vital stain exclusion, is high for at least one month. Trabecular ultrastructure, as compared to that of fresh eyes, first shows minor cellular and extracellular matrix degradation after 3 weeks in culture. The biosynthetic profiles of trabecular glycosaminoglycans (GAGs) change significantly by 3 weeks in culture. Eyes that are stored at 5°C for up to 48 hr postmortem exhibit changes in trabecular ultrastructure and in GAG profiles; both characteristics return to normal by 7 days in culture. The incorporation pattern of 35S-sulfate and 3H-glucosamine into the GAGs of the trabecular meshwork (TM) is distinct from corneal or scleral incorporation. The relative incorporation of 3H-glucosamine into trabecular GAGs, as determined by sequential enzy- matic degradation, is: 22.3% hyaluronic acid (HA), 27.9% chondroitin sulfate (CS), 21.3% dermatan sulfate (DS), 5.9% keratan sulfate (KS), 17.7% heparan sulfate (HS) and 4.9% unidentified material. The relative incorporation of 35S-sulfate into trabecular GAGs is: 0% HA, 32.9% CS, 34.8% DS, 7.7% KS, 13.8% HS and 11.1% into unidentified material. This profile is in good agreement with the profile that was previously obtained for human and nonhuman primate meshworks prior to culture. -
The Development of the Trabecular Meshwork and Its Abnormality in Primary- Infantile Glaucoma*
THE DEVELOPMENT OF THE TRABECULAR MESHWORK AND ITS ABNORMALITY IN PRIMARY- INFANTILE GLAUCOMA* BY Douglas R. Anderson, MD INTRODUCTION IN CONGENITAL GLAUCOMA, THE PERIPHERAL IRIS IS SEEN BY GONIOSCOPY TO BE inserted more anteriorly than normal, and the trabecular meshwork seems to have a shiny surface. 1-4 At the time of goniotomy, this surface tissue is incised, and the peripheral iris falls posteriorly as if the incised tissue had been holding the iris insertion forward. Since the operation usually relieves the elevated pressure,4 it is natural to assume that the shiny surface is an imperforate membrane that had prevented aqueous humor outflow. Histopathologic confirmation of this presumed membrane or other pathologic features typical of infantile glaucoma has been difficult to obtain. Specimens enucleated after long-standing severe glaucoma, per- haps after several surgical procedures, contain secondary changes that hide the nature ofthe initial abnormality. Other infantile glaucoma speci- mens were obtained at autopsy from infants who died of associated fatal congenital anomalies. The abnormalities in these eyes may not be the same as the abnormality that produces primary infantile glaucoma in otherwise normal infants. Furthermore, most investigators have not clari- fied which morphologic features of the angles of infantile glaucomatous eyes are characteristics of infant eyes (known to differ from adult eyes *From the Bascom Palmer Eye Institute, Department of Ophthalmology, University of Miami School of Medicine, Miami, Florida. This investigation was supported in part by Public Health Service Research Grant EY-00031 from the National Eye Institute, Bethesda, Maryland, by the Glaucoma Re- search Fund of the American Health Assistance Foundation, by the John Russell Stubbins Foundation, and by the Phillips Foundation. -
Targeting the Trabecular Meshwork, Schlemm's Canal and the Collector Channels
WHITEPAPER Targeting the Trabecular Meshwork, Schlemm’s Canal and the Collector Channels The Evolution of Canaloplasty and the iTrack™ Canaloplasty Microcatheter As our understanding of the pathogenesis of glaucoma has evolved, the role of the proximal versus distal portions of the conventional outflow pathway as therapeutic targets has generated growing interest. It is well understood that the trabecular meshwork, specifically the extracellular matrix filling the open space of the juxtacanalicular connective tissue (JCT) is the primary site of outflow resistance in the conventional outflow pathway.1 It is also known that glaucomatous pathology can cause significant outflow resistance in distal pathways.2 Greater contractility of Schlemm’s canal has been shown to increase outflow resistance.3,4,5 Further, the canal in eyes with primary open angle glaucoma (POAG) tends to be shorter, narrowed, and often collapsed, reducing the area of active flow.3,4,5 Another significant cause of increased outflow resistance in POAG eyes is herniations of the trabecular meshwork obstructing up to 90% of collector channels.6 Armed with improved Introduced in 2005 and approved First conceived through an understanding of the function by the FDA in 2008, canaloplasty ab-externo approach as an ™ and physiology of the proximal with the iTrack microcatheter alternative to trabeculectomy, has evolved to be a procedure surgeons have developed and distal outflow pathways in with high utility in the treatment an ab-interno canaloplasty glaucomatous eyes, an increasing of glaucoma. It is indicated in a technique that is conjunctiva number of surgeons are turning wide variety of glaucoma types and sclera sparing and thus to ab-interno canaloplasty to including; POAG, pigmentary can be deployed earlier in the target each segment of the (PG), pseudoexfoliation (PXF) disease process. -
Regenerative Capacity of the Corneal Transition Zone for Endothelial Cell Therapy
Sie et al. Stem Cell Research & Therapy (2020) 11:523 https://doi.org/10.1186/s13287-020-02046-2 REVIEW Open Access Regenerative capacity of the corneal transition zone for endothelial cell therapy Nicole Ming Sie1,2, Gary Hin-Fai Yam1,3* , Yu Qiang Soh1,2, Matthew Lovatt1, Deepinder Dhaliwal3, Viridiana Kocaba1,4 and Jodhbir S. Mehta1,2,5,6* Abstract The corneal endothelium located on the posterior corneal surface is responsible for regulating stromal hydration. This is contributed by a monolayer of corneal endothelial cells (CECs), which are metabolically active in a continuous fluid-coupled efflux of ions from the corneal stroma into the aqueous humor, preventing stromal over- hydration and preserving the orderly arrangement of stromal collagen fibrils, which is essential for corneal transparency. Mature CECs do not have regenerative capacity and cell loss due to aging and diseases results in irreversible stromal edema and a loss of corneal clarity. The current gold standard of treatment for this worldwide blindness caused by corneal endothelial failure is the corneal transplantation using cadaveric donor corneas. The top indication is Fuchs corneal endothelial dystrophy/degeneration, which represents 39% of all corneal transplants performed. However, the global shortage of transplantable donor corneas has restricted the treatment outcomes, hence instigating a need to research for alternative therapies. One such avenue is the CEC regeneration from endothelial progenitors, which have been identified in the peripheral endothelium and the adjacent transition zone. This review examines the evidence supporting the existence of endothelial progenitors in the posterior limbus and summarizes the existing knowledge on the microanatomy of the transitional zone. -
Pathology of the Glaucomas
146 Br J Ophthalmol: first published as 10.1136/bjo.56.3.146 on 1 March 1972. Downloaded from Pathology of the glaucomas DOUGLAS R. ANDERSON From the Bascom Palmer Eye Institute, Department of Ophthalmology, University of Miami School of Medicine, Miami, Florida, U.S.A. It is hoped that this review ofpathological mechanisms in glaucoma will provide a basis for discussion by pointing out matters of which we are still ignorant and by challenging some of the currently accepted concepts. It is convenient to divide considerations of pathology into two parts: (i) The cause of the pressure elevation. (2) The ocular damage resulting from raised intraocular pressure. Cause of elevated intraocular pressure PRIMARY OPEN-ANGLE GLAUCOMA In the normal eye, the main resistance to aqueous outflow is in the outermost region of the trabecular meshwork, which is adjacent to and forms the inner wall of Schlemm's canal.copyright. In primary open-angle glaucoma, there is increased resistance to outflow, and we assume that the abnormal additional resistance is in the same location. Examinations of patho- logical anatomy show scarring and sclerosis in the trabecular meshwork, but the specimens are usually taken from advanced cases and it has not been decided to what degree trabecular changes may be due to damage secondary to long-standing elevated intraocular pressure rather than the primary defect. Electron microscopy, which Dr. Tripathi will cover in http://bjo.bmj.com/ detail, shows fewer vacuoles in the endothelium of Schlemm's canal in patients with glaucoma. Again, it is not clear whether the fewer vacuoles account for diminished outflow facility or whether they merely reflect alterations in flow dynamics because of resistance elsewhere in the outflow pathway. -
(12) United States Patent (10) Patent No.: US 8,684,743 B2 Van Dalen Et Al
USOO8684743B2 (12) United States Patent (10) Patent No.: US 8,684,743 B2 Van Dalen et al. (45) Date of Patent: Apr. 1, 2014 (54) MODEL HUMAN EYE AND FACE MANIKIN 5, 195,896 A 3/1993 Sweeney et al. FOR USE THEREWITH 5,561,137 A 10, 1996 Or et al. 5,893,719 A 4/1999 Radow (75) Inventors: Johan T. W. Van Dalen, Tucson, AZ (Continued) (US); Dan D. Carda, Tucson, AZ (US) FOREIGN PATENT DOCUMENTS (73) Assignee: Eye Care and Cure Pte. Ltd, Singapore (SG) EP 1193664 A2 4/2002 JP 2007 127708 A 5/2007 (*) Notice: Subject to any disclaimer, the term of this WO 2009 152582 A1 12/2009 patent is extended or adjusted under 35 OTHER PUBLICATIONS U.S.C. 154(b) by 299 days. Gray,ray, AnatomyaOW ofO the h Ula bodyO 20,, sectionSec1On 1 C.I.c.1. The TUCS O f (21) Appl. No.: 13/189,434 the Eye), 1918, Retreived from http://www.bartleby.com/107/225. html. (22) Filed: Jul. 22, 2011 (Continued)O1 (65) Prior Publication Data Primary Examiner — Benjamin Layno US 2012/OO21397 A1 Jan.• 1-ys26, 2012 74). A ttorney,ey, Agent,Ag or FFirm — Dale F. Regelman;Regel Quarles1 Related U.S. Application Data & Brady LLP (60) Provisional application No. 61/367,335, filed on Jul. (57) ABSTRACT 23, 2010. A model human eye that is structurally Suited for practicing Surgical techniques, including extraocular muscle resection (51) G09BInt. Cl. 23/30 2006.O1 and recession, is presented. The model eye comprises a hemi ( .01) spherical-shaped, bottom assembly having multiple retinal (52) U.S.