Titre Du Document
Total Page:16
File Type:pdf, Size:1020Kb
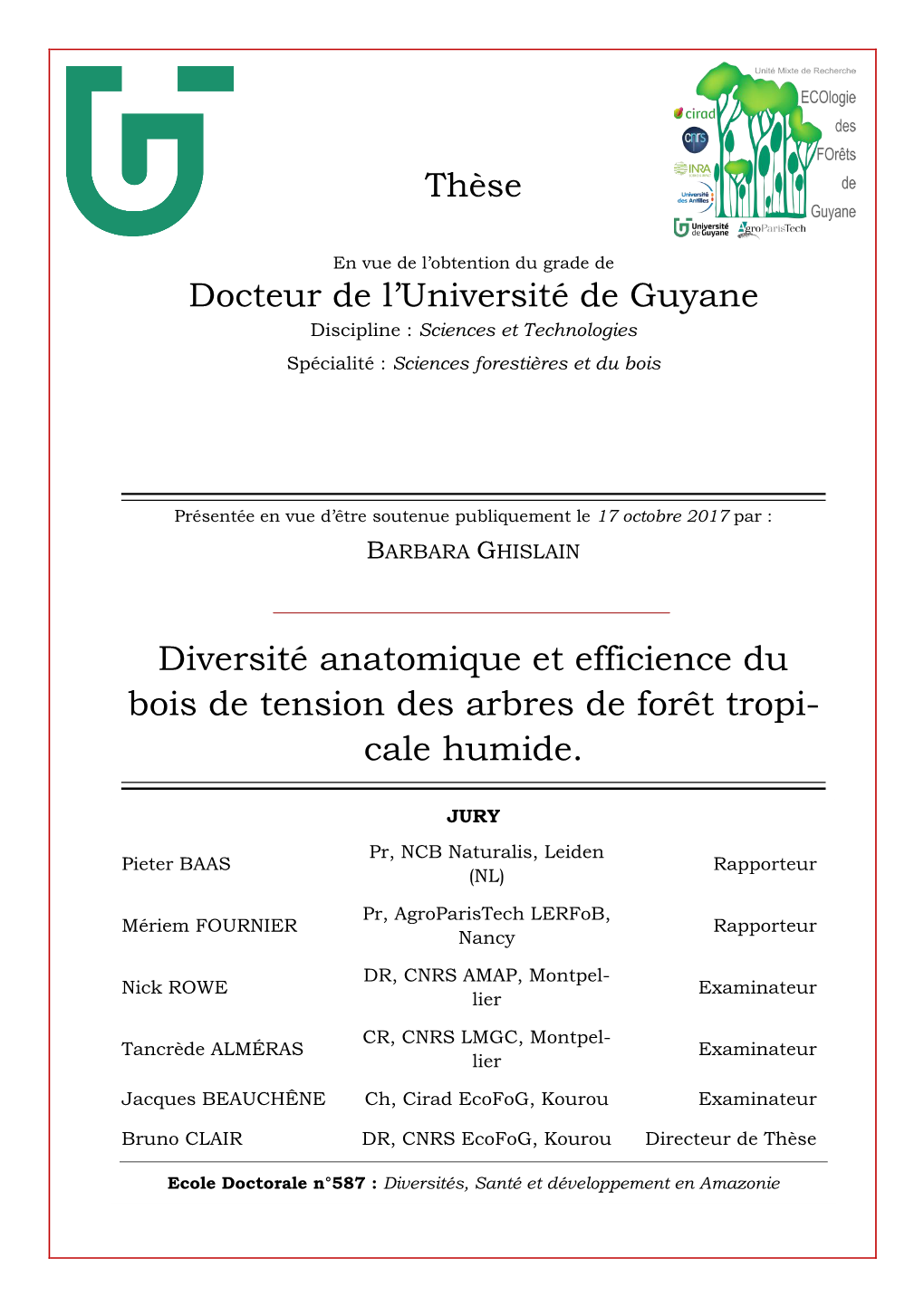
Load more
Recommended publications
-
Tree Composition and Ecological Structure of Akak Forest Area
Environment and Natural Resources Research; Vol. 9, No. 4; 2019 ISSN 1927-0488 E-ISSN 1927-0496 Published by Canadian Center of Science and Education Tree Composition and Ecological Structure of Akak Forest Area Agbor James Ayamba1,2, Nkwatoh Athanasius Fuashi1, & Ayuk Elizabeth Orock1 1 Department of Environmental Science, University of Buea, Cameroon 2 Ajemalebu Self Help, Kumba, South West Region, Cameroon Correspondence: Agbor James Ayamba, Department of Environmental Science, University of Buea, Cameroon. Tel: 237-652-079-481. E-mail: [email protected] Received: August 2, 2019 Accepted: September 11, 2019 Online Published: October 12, 2019 doi:10.5539/enrr.v9n4p23 URL: https://doi.org/10.5539/enrr.v9n4p23 Abstract Tree composition and ecological structure were assessed in Akak forest area with the objective of assessing the floristic composition and the regeneration potentials. The study was carried out between April 2018 to February 2019. A total of 49 logged stumps were selected within the Akak forest spanning a period of 5 years and 20m x 20m transects were demarcated. All plants species <1cm and above were identified and recorded. Results revealed that a total of 5239 individuals from 71 families, 216 genera and 384species were identified in the study area. The maximum plants species was recorded in the year 2015 (376 species). The maximum number of species and regeneration potentials was found in the family Fabaceae, (99 species) and (31) respectively. Baphia nitida, Musanga cecropioides and Angylocalyx pynaertii were the most dominant plants specie in the years 2013, 2015 and 2017 respectively. The year 2017 depicts the highest Simpson diversity with value of (0.989) while the year 2015 show the highest Simpson dominance with value of (0.013). -
PHYTOCHEMICALS and BIOACTIVITIES of Garcinia Prainiana KING and G
PHYTOCHEMICALS AND BIOACTIVITIES OF Garcinia prainiana KING AND G. hombroniana PIERRE SHAMSUL ON A thesis submitted in fulfilment of the requirements for the award of the degree of Doctor of Philosophy (Chemistry) Faculty of Science Universiti Teknologi Malaysia MARCH 2018 iii To My Beloved Wife Najatulhayah Alwi and My children, Muhd Nabil AnNajat, Muhd Nabihan AnNajat, Muhd Naqib AnNajat, Muhd Nazeem AnNajat, Shahmina Nasyamah AnNajat For Their Love, Support and Best Wishes. iv ACKNOWLEDGEMENT First and foremost, I show my gratitude to The Almighty God for giving me the strength to complete this thesis. I am deeply grateful to everyone who has helped me in completing this work. Thanks a million to my supervisor, Assoc. Prof. Dr. Farediah Ahmad, Prof. Dr. Hasnah Mohd Sirat and Assoc. Prof. Dr. Muhammad Taher for their untiring assistance, direction, encouragement, comments, suggestions, enthusiasm, continuous guidance, ideas, constructive criticism and unrelenting support throughout this work. I would like to thank the Department of Chemistry, Faculty of Science, UTM for the access of UV, IR, GC-MS, and NMR instruments. Sincerely thanks to all lab assistants especially to Mr. Azmi, Mr. Rashidi, Mr. Amin and Mr. Hairol for their help throughout these seven years. Special thanks to my lab mates; Wan Muhd Nuzul, Athirah, Salam, Aminu, Saidu, Shariha, Awanis, Iman, Erni, Edelin, Suri and Yani for their moral support, advice and encouragement to make the lab work meaningful. I am grateful to staff scholarship by Ministry of Higher Education for my doctoral fellowship and Research University Grant (GUP), Universiti Teknologi Malaysia under vote 03H93 for the support throughout the entire research. -
The Diversity of Conservation: Exploring Narratives, Relationships and Ecosystem Services in Melanesian Market-Based Biodiversity Conservation
THE DIVERSITY OF CONSERVATION: EXPLORING NARRATIVES, RELATIONSHIPS AND ECOSYSTEM SERVICES IN MELANESIAN MARKET-BASED BIODIVERSITY CONSERVATION A DISSERTATION SUBMITTED TO THE FACULTY OF THE UNIVERSITY OF MINNESOTA BY BRIDGET M. HENNING IN PARTIAL FULFILLMENT OF THE REQUIREMENTS FOR THE DEGREE OF DOCTOR OF PHILOSOPHY DR. DAVID LIPSET, CO-ADVISOR & DR. GEORGE WEIBLEN, CO-ADVISOR OCTOBER 2014 © Bridget M. Henning 2014 Acknowledgements I am endlessly grateful to the Sogeram River communities for their cooperation, assistance, and friendship, especially the Wanang community, which took me in as their own. For their hospitality, I would like to thank Filip Damen and Maria Sepu in Wanang, Paul Mansa in Palimul, Paul and Evelyn Hangre in Munge, Catherine and Benny in Manimagi, John and Miagi in Tiklik, and Christina Sepu in Wagai. I would like to thank Clara and Yolli Agigam for helping me to learn Tok Pisin and easing my transition to village life. I appreciate the time and patience Filip Damen, Jepi Rop, Albert and Samuel Mansa, Samson Mareks, Mak Mulau, and Jori Umbang put towards teaching me about conservation. Thank you to Raymond Kuam for looking after me and to Manuel for always making sure I had enough to eat. I am indebted to the women who helped me learn to live in Wanang and taught me what it was to be good kin, especially Clara and Katie Sebo, Mugunas, Joyce, and Clara Filip, Anna Jori, Anna Sothan, Rosa Samson, Doris Samuel, Polina Nambi, and Samaras Ukiem. Special thanks to Maria Sepu for being a truly amazing woman and wonderful friend. I would like to thank the New Guinea Binatang Research Center especially Vojtech Novotny, Marcus Manumbor, Martin Mogia, Gibson Sosanika, Hans Nowatuo, Elvis Tamtiai, and Joanne Kavagu for logistical and moral support and for patiently explaining Melanesian conservation. -
Terrestrial Biodiversity Field Assessment in the May River and Upper Sepik River Catchments SDP-6-G-00-01-T-003-018
Frieda River Limited Sepik Development Project Environmental Impact Statement Appendix 8b – Terrestrial Biodiversity Field Assessment in the May River and Upper Sepik River Catchments SDP-6-G-00-01-T-003-018 Terrestrial Biodiversity Field Assessment in the May River and Upper Sepik River Catchments Sepik Development Project (Infrastructure Corridor) August 2018 SDP-6-G-00-01-T-003-018 page i CONTRIBUTORS Wayne Takeuchi Wayne is a retired tropical forest research biologist from the Harvard University Herbaria and Arnold Arboretum. He is one of the leading floristicians in Papuasian botany and is widely known in professional circles for wide-ranging publications in vascular plant taxonomy and conservation. His 25-year career as a resident scientist in Papua New Guinea began in 1988 at the Wau Ecology Institute (subsequently transferring to the PNG National Herbarium in 1992) and included numerous affiliations as a research associate or consultant with academic institutions, non-governmental organisations (NGOs) and corporate entities. Despite taking early retirement at age 57, botanical work has continued to the present on a selective basis. He has served as the lead botanist on at least 38 multidisciplinary surveys and has 97 peer-reviewed publications on the Malesian flora. Kyle Armstrong, Specialised Zoological Pty. Ltd – Mammals Dr Kyle Armstrong is a consultant Zoologist, trading as ‘Specialised Zoological’, providing a variety of services related to bats, primarily on acoustic identification of bat species from echolocation call recordings, design and implementation of targeted surveys and long term monitoring programmes for bats of conservation significance, and the provision of management advice on bats. He is also currently Adjunct Lecturer at The University of Adelaide, an Honorary Research Associate of the South Australian Museum, and had four years as President of the Australasian Bat Society, Inc. -
Acetolysed Thin Layer Of
Pollen morphology of the genus Hydnocarpus (Flacourtiaceae) with notes on related genera J. Schaeffer Rijksherbarium, Leiden Contents Summary 65 I Introduction 65 II General morphology 66 III Systematic descriptions 66 IV Pollen types 76 Pollen and V morphology taxonomy 77 References 79 Summary Pollen of ofthe described. Two grains 34 species genus Hydnocarpus(Flacourtiaceae) are pollen types, one of which is subdivided in two subtypes, are distinguished. Within Flacourtiaceae the pollen of Hydnocarpus In is more or less isolated, but the related genus Chlorocarpa has rather similar pollen. sculpture there exists some resemblance to Paropsia (Passifloraceae). I. Introduction The present study forms part of a general pollenmorphological survey of the family Flacourtiaceae, initiated at the Rijksherbarium in collaboration with Dr. H. Sleumer. The selected for the first detailed because genus Hydnocarpus was study it proved to have rather characteristic pollen types and is concentrated in SE. Asia and the Malesian region. Moreover, an adequate amountof well-determinedmaterial as well as a recent revision ot In the genus (Sleumer, 1954) were available. total 34 species could be investigated, of the total numberof known. representing 85 % species In addition, the pollen of a large other number of Flacourtiaceae was cursorily examined, in order to asses the pollen of within the morphological relationships Hydnocarpus family. The pollen of the genera and which resemble that of described Neoptychocarpus Chlorocarpa, Hydnocarpus most, is in more detail. Erdtman described Previously, only (1952) has, very briefly, the pollen of Hydnocarpus rather the elmeri which, however, proved to be atypical of genus. The pollen material was acetolysed for two minutes, mounted in glycerin jelly and photographed with a Leitz apochromatic OI objective (90/1.40). -
Curriculum Vitae
CURRICULUM VITAE Paul Edward Berry Current address: Dr. Paul E. Berry, Department of Ecology and Evolutionary Biology, 2035 Kraus Natural Science Bldg, 830 N University Avenue, Ann Arbor, MI 48109-1048 -and- University Herbarium, 3600 Varsity Drive, Ann Arbor, MI 48108-2287 Office phone: 734-647-3689 Fax: 734-763-0544 email: [email protected] Education and Personal Data Born in Boston, Massachusetts, USA B.S., Biology, Haverford College, Haverford, Pennsylvania, 1975 M.A., Biology, Washington University, St. Louis, Missouri, 1979 Ph.D., Biology, Washington University, St. Louis, Missouri, 1980 Employment and Professional History 2006– University of Michigan–Ann Arbor: Professor, Department of Ecology and Evolutionary Biology; Curator, UM Herbarium 2006–15 University of Michigan–Ann Arbor: Director of University Herbarium 2007-08 Interim Director, Matthaei Botanical Gardens and Nichols Arboretum, University of Michigan–Ann Arbor 1998–2005 University of Wisconsin–Madison: Associate then full Professor and Herbarium Director, Botany Dept.; Core Faculty member, Latin American, Caribbean, and Ibero-American Studies Program; Faculty Associate, Institute for Environmental Studies 1989–97 Missouri Botanical Garden, St. Louis, Missouri: Curator; Adjunct Associate Professor at Washington University, St. Louis (1995-98) and Adjunct Associate Professor at University of Missouri–St. Louis (1989-97) 1980–88 Universidad Simón Bolívar, Caracas, Venezuela: Assistant then Associate Professor, Departamento de Biología de Organismos, (Department Chair, 1984– 86) 1978–79 Washington University, St. Louis: Teaching Assistant 1975–76 Comisión para el Desarrollo del Sur de Venezuela (CODESUR), Ministerio de Obras Públicas: Botanist Research Interests and Other Activities Plant systematics, phytogeography, and floristics. Taxonomic focus on large genera in the Euphorbiaceae (Croton and Euphorbia); Fuchsia (Onagraceae); Rapateaceae. -
Aporosa Blume from the Paleoequatorial Rainforest of Bikaner, India: Its Evolution and Diversification in Deep Time Journal Item
Open Research Online The Open University’s repository of research publications and other research outputs Aporosa Blume from the paleoequatorial rainforest of Bikaner, India: Its evolution and diversification in deep time Journal Item How to cite: Shukla, Anumeha; Mehrotra, Rakesh C.; Spicer, Robert A. and Spicer, Teresa E.V. (2016). Aporosa Blume from the paleoequatorial rainforest of Bikaner, India: Its evolution and diversification in deep time. Review of Palaeobotany and Palynology, 232 pp. 14–21. For guidance on citations see FAQs. c 2017 Elsevier B.V. https://creativecommons.org/licenses/by-nc-nd/4.0/ Version: Accepted Manuscript Link(s) to article on publisher’s website: http://dx.doi.org/doi:10.1016/j.revpalbo.2016.05.006 Copyright and Moral Rights for the articles on this site are retained by the individual authors and/or other copyright owners. For more information on Open Research Online’s data policy on reuse of materials please consult the policies page. oro.open.ac.uk ÔØ ÅÒÙ×Ö ÔØ Aporosa Blume from the paleoequatorial rainforest of Bikaner, India: its evolution and diversification in deep time Anumeha Shukla, Rakesh C. Mehrotra, Robert A. Spicer, Teresa E.V. Spicer PII: S0034-6667(16)30093-8 DOI: doi: 10.1016/j.revpalbo.2016.05.006 Reference: PALBO 3756 To appear in: Review of Palaeobotany and Palynology Received date: 23 July 2015 Revised date: 11 May 2016 Accepted date: 27 May 2016 Please cite this article as: Shukla, Anumeha, Mehrotra, Rakesh C., Spicer, Robert A., Spicer, Teresa E.V., Aporosa Blume from the paleoequatorial rainforest of Bikaner, In- dia: its evolution and diversification in deep time, Review of Palaeobotany and Palynology (2016), doi: 10.1016/j.revpalbo.2016.05.006 This is a PDF file of an unedited manuscript that has been accepted for publication. -
AKTIVITAS ANTIOKSIDAN DAN ANTIKANKER SENYAWA GARCINOL DARI EKSTRAK N-HEKSANA KULIT BATANG Garcinia Maingayi Hook
AKTIVITAS ANTIOKSIDAN DAN ANTIKANKER SENYAWA GARCINOL DARI EKSTRAK N-HEKSANA KULIT BATANG Garcinia maingayi Hook SKRIPSI LUCYTA SARI PROGRAM STUDI KIMIA FAKULTAS SAINS DAN TEKNOLOGI UNIVERSITAS ISLAM NEGERI SYARIF HIDAYATULLAH JAKARTA 2018 M/ 1440 H AKTIVITAS ANTIOKSIDAN DAN ANTIKANKER SENYAWA GARCINOL DARI EKSTRAK N-HEKSANA KULIT BATANG Garcinia maingayi Hook SKRIPSI Sebagai Salah Satu Syarat untuk Memperoleh Gelar Sarjana Sains Program Studi Kimia Fakultas Sains dan Teknologi Universitas Islam Negeri Syarif Hidayatullah Jakarta Oleh: LUCYTA SARI 11140960000068 PROGRAM STUDI KIMIA FAKULTAS SAINS DAN TEKNOLOGI UNIVERSITAS ISLAM NEGERI SYARIF HIDAYATULLAH JAKARTA 2018 M/ 1440 H ABSTRAK LUCYTA SARI. Aktivitas Antioksidan dan Antikanker Senyawa Garcinol dari Ekstrak n-Heksana Kulit Batang Garcinia maingayi Hook. Dibimbing oleh SRI HARTATI dan SITI NURBAYTI Garicinia maingayi Hook merupakan salah satu tanaman obat yang mengandung senyawa xanton, benzofenon, dan triterpenoid yang memiliki aktivitas antioksidan dan antikanker. Uji pendahuluan terhadap ekstrak n-heksana kulit batang G. maingayi menunjukkan aktivitas antioksidan dan antikanker payudara (MCF-7) berturut-turut sebesar 90,51% (100 μg/mL) dan 96,87% (200 μg/mL). Penelitian lanjutan ini dilakukan untuk mengidentifikasi struktur senyawa murni ekstrak n-heksana kulit batang G. maingayi dan menguji aktivitasnya. Isolasi dan fraksinasi senyawa murni dilakukan dengan metode kromatografi kolom. Uji aktivitas antioksidan dan antikanker dilakukan menggunakan metode DPPH (2,2- difenil-1-pikrilhidrazil) dan MTT [3-(4,5-dimetiltiazol-2-il)-2,5-difeniltetrazolium bromida]. Penentuan struktur senyawa menggunakan metode spektroskopi. Isolat GM-1 yang diperoleh berupa kristal jarum kuning pucat dengan titik leleh 122- 124˚C. Analisis isolat GM-1 menggunakan UV-Vis menunjukkan karakteristik senyawa golongan benzofenon dengan λmax 251 nm (kromofor karbonil) dan λmax 355 nm (kromofor aromatik). -
Wild Edible Fruits of Colombia
Wild edible fruits of Colombia: diversity and use prospects Frutos silvestres comestibles de Colombia: diversidad y perspectivas de uso Diana López Diago , Néstor García Abstract Wild fruits have been an integral part of the diet of rural inhabitants in tropical America. In Colombia, information on the use of wild fruits appears scattered in the ethnobotanical literature and herbaria collections, limiting the design of conservation and use strategies. This review aims to synthesize information about the wild fruit species used in Colombia. We reviewed herbarium collections and literature references. We recorded 703 species in 76 families, among which Fabaceae (66 species), Arecaceae (58), and Passifloraceae (44) were the most diverse. The genera with more species were Inga (42), Passiflora (42), and Pouteria (21). Most species are widely distributed in tropical America, and only 45 (6.4 %) are endemic to Colombia. The regions with the largest number of species were the Amazon (388), Andes (144), and Pacific (111). Most of the recorded species, 613 (87.2 %), are exclusively wild, whereas 90 (12.8 %) are wild or cultivated. Wild edible fruits have a high potential for agriculture, novel products and nutritional improvement; however, it is vital to create strategies to revalorize their use. Keywords. Biodiversity. Ethnobotany. Underutilized species. Wild foods. Resumen Los frutos silvestres han sido una parte integral de la dieta de los habitantes rurales del trópico americano. En Colombia, la información acerca del uso de los frutos silvestres se encuentra dispersa en la literatura etnobotánica y en colecciones de herbario, limitando el diseño de estrategias de conservación y uso. Esta revisión tiene como propósito sintetizar información acerca de los frutos silvestres usados en Colombia. -
A New Miocene Malpighialean Tree from Panama
Rodriguez-ReyesIAWA Journal et al. – New38 (4), Miocene 2017: malpighialean437–455 wood 437 Panascleroticoxylon crystallosa gen. et sp. nov.: a new Miocene malpighialean tree from Panama Oris Rodriguez-Reyes1, 2, Peter Gasson3, Carolyn Thornton4, Howard J. Falcon-Lang5, and Nathan A. Jud6 1Smithsonian Tropical Research Institute, Box 0843-03092, Balboa, Ancón Republic of Panamá 2Facultad de Ciencias Naturales, Exactas y Tecnología, Universidad de Panamá, Apartado 000 17, Panamá 0824, Panamá 3Jodrell Laboratory, Royal Botanic Gardens, Kew, Richmond, Surrey TW9 3DS, United Kingdom 4Florissant Fossil Beds National Monument, P.O. Box 185, 15807 Teller County Road 1, Florissant, CO 80816, U.S.A. 5Department of Earth Sciences, Royal Holloway, University of London, Egham, Surrey TW20 0EX, United Kingdom 6L.H. Bailey Hortorium, Department of Plant Biology, 412 Mann Library Building, Cornell University, Ithaca, NY 14853, U.S.A. *Corresponding author; e-mail: [email protected] ABSTRACT We report fossil wood specimens from two Miocene sites in Panama, Central America: Hodges Hill (Cucaracha Formation; Burdigalian, c.19 Ma) and Lago Alajuela (Alajuela Formation; Tortonian, c.10 Ma), where material is preserved as calcic and silicic permineralizations, respectively. The fossils show an unusual combination of features: diffuse porous vessel arrangement, simple perforation plates, alternate intervessel pitting, vessel–ray parenchyma pits either with much reduced borders or similar to the intervessel pits, abundant sclerotic tyloses, rays markedly heterocellular with long uniseriate tails, and rare to absent axial parenchyma. This combination of features allows assignment of the fossils to Malpighiales, and we note similarities with four predominantly tropical families: Salicaceae, Achariaceae, and especially, Phyllanthaceae, and Euphorbiaceae. -
Smithsonian Plant Collections, Guyana 1995–2004, H
Smithsonian Institution Scholarly Press smithsonian contributions to botany • number 97 Smithsonian Institution Scholarly Press ASmithsonian Chronology Plant of MiddleCollections, Missouri Guyana Plain s 1995–2004,Village H. David Sites Clarke By Craig M. Johnson Carol L. Kelloff, Sara N. Alexander, V. A. Funk,with contributions and H. David by Clarke Stanley A. Ahler, Herbert Haas, and Georges Bonani SERIES PUBLICATIONS OF THE SMITHSONIAN INSTITUTION Emphasis upon publication as a means of “diffusing knowledge” was expressed by the first Secretary of the Smithsonian. In his formal plan for the Institution, Joseph Henry outlined a program that included the following statement: “It is proposed to publish a series of reports, giving an account of the new discoveries in science, and of the changes made from year to year in all branches of knowledge.” This theme of basic research has been adhered to through the years by thousands of titles issued in series publications under the Smithsonian imprint, com- mencing with Smithsonian Contributions to Knowledge in 1848 and continuing with the following active series: Smithsonian Contributions to Anthropology Smithsonian Contributions to Botany Smithsonian Contributions to History and Technology Smithsonian Contributions to the Marine Sciences Smithsonian Contributions to Museum Conservation Smithsonian Contributions to Paleobiology Smithsonian Contributions to Zoology In these series, the Institution publishes small papers and full-scale monographs that report on the research and collections of its various museums and bureaus. The Smithsonian Contributions Series are distributed via mailing lists to libraries, universities, and similar institu- tions throughout the world. Manuscripts submitted for series publication are received by the Smithsonian Institution Scholarly Press from authors with direct affilia- tion with the various Smithsonian museums or bureaus and are subject to peer review and review for compliance with manuscript preparation guidelines. -
Multilayered Structure of Tension Wood Cell Walls in Salicaceae Sensu Lato
Multilayered structure of tension wood cell walls in Salicaceae sensu lato and its taxonomic significance Barbara Ghislain, Eric-André Nicolini, Raïssa Romain, Julien Ruelle, Arata Yoshinaga, Mac H. Alford, Bruno Clair To cite this version: Barbara Ghislain, Eric-André Nicolini, Raïssa Romain, Julien Ruelle, Arata Yoshinaga, et al.. Mul- tilayered structure of tension wood cell walls in Salicaceae sensu lato and its taxonomic significance. Botanical Journal of the Linnean Society, Linnean Society of London, 2016, 182 (4), pp.744-756. 10.1111/boj.12471. hal-01392845 HAL Id: hal-01392845 https://hal.archives-ouvertes.fr/hal-01392845 Submitted on 4 Nov 2016 HAL is a multi-disciplinary open access L’archive ouverte pluridisciplinaire HAL, est archive for the deposit and dissemination of sci- destinée au dépôt et à la diffusion de documents entific research documents, whether they are pub- scientifiques de niveau recherche, publiés ou non, lished or not. The documents may come from émanant des établissements d’enseignement et de teaching and research institutions in France or recherche français ou étrangers, des laboratoires abroad, or from public or private research centers. publics ou privés. Multilayered structure of tension wood cell walls in Salicaceae sensu lato and its taxonomic significance Barbara Ghislain1*, Eric-André Nicolini2, Raïssa Romain1, Julien Ruelle3, Arata Yoshinaga4, Mac H. Alford5, Bruno Clair1 1 CNRS, UMR EcoFoG, AgroParisTech, Cirad, INRA, Université des Antilles, Université de Guyane, 97310 Kourou, France 2 CIRAD, AMAP, botAnique et bioinforMatique de l’Architecture des Plantes, Campus Agronomique BP 701, 97387 Kourou, French Guiana, France 3 INRA, Laboratoire d’Etude des Ressources Forêt-Bois (LERFoB), 54280 Champenoux, Nancy, France 4 Laboratory of Tree Cell Biology, Graduate School of Agriculture, Kyoto University, Sakyo- ku, Kyoto 606-8502, Japan 5 Department of Biological Sciences, University of Southern Mississippi, 118 College Drive #5018, Hattiesburg, Mississippi 39406, U.S.A.