Trabecular Bone Tissue of the Wrist Bones in Primates
Total Page:16
File Type:pdf, Size:1020Kb
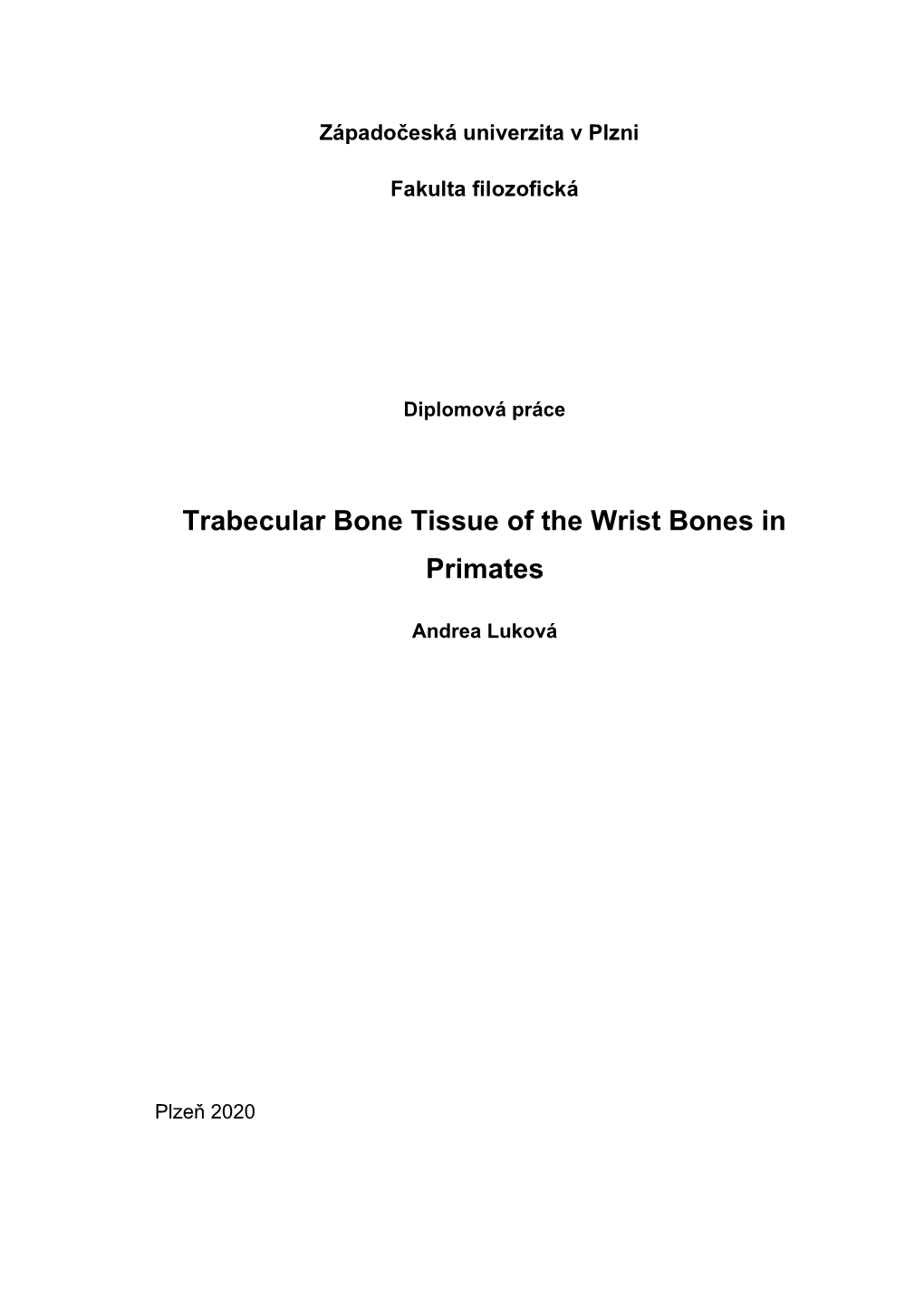
Load more
Recommended publications
-
Sergio Almécija
Sergio Almécija Center for the Advanced Study of Human Paleobiology Email: [email protected] Department of Anthropology Cellphone: (646) 943-1159 The George Washington University Science and Engineering Hall 800 22nd Street NW, Suite 6000 Washington, DC 20052 EDUCATION PhD, Cum Laude. Institut Català de Paleontologia Miquel Crusafont at Universitat Autònoma de Barcelona and Universitat de Barcelona, Biological Anthropology, (October 30th, 2009). Dissertation: Evolution of the hand in Miocene apes: implications for the appearance of the human hand. Advisor: Salvador Moyà-Solà. MA with Advanced Studies Certificate (DEA). Institut Català de Paleontologia Miquel Crusafont at Universitat Autònoma de Barcelona. Biological Anthropology, 2007. BS. Universitat Autònoma de Barcelona, Biological Sciences, 2005. PROFESSIONAL APPOINTMENTS Assistant Professor. Center for the Advanced Study of Human Paleobiology, Department of Anthropology, The George Washington University. Present. Research Instructor. Department of Anatomical Sciences, Stony Brook University. 2012-2015. Fulbright Postdoctoral Fellow. Department of Vertebrate Paleontology, American Museum of Natural History and New York Consortium in Evolutionary Primatology. 2010-2012. Research Associate. Department of Paleoprimatology and Human Paleontology, Institut Català de Paleontologia Miquel Crusafont. 2010-present. RESEARCH INTERESTS Evolution of humans and apes. Based on the morphology of living and fossil hominoids (and other primates), to identify key skeletal adaptations defining different stages of great ape and human evolution, as well as the original selective pressures responsible for specific evolutionary transitions. Morphometrics. Apart from describing new great ape and hominin fossil materials, I am interested in broad comparative studies of key regions of the skeleton using state-of-the-art methods such as three-dimensional morphometrics and phylogenetically-informed comparative methods. -
For Peer Review
View metadata, citation and similar papers at core.ac.uk brought to you by CORE Page 1 of 57 Journal of Morphology provided by Archivio della Ricerca - Università di Pisa 1 2 3 Title: The locomotion of Babakotia radofilai inferred from epiphyseal and diaphyseal 4 5 6 morphology of the humerus and femur. 7 8 9 Damiano Marchi1,2*, Christopher B. Ruff3, Alessio Capobianco, 1,4, Katherine L. Rafferty5, 10 11 Michael B. Habib6, Biren A. Patel2,6 12 13 14 1 15 Department of Biology, University of Pisa, Pisa, Italy, 56126 16 17 2 18 Evolutionary Studies Institute, University of the Witwatersrand, Johannesburg, South 19 20 Africa, WITS 2050 For Peer Review 21 22 23 3 Center for Functional Anatomy and Evolution, Johns Hopkins University School of 24 25 26 Medicine, Baltimore, MD 21111 27 28 4 29 Scuola Normale Superiore, Pisa, Italy, 56126 30 31 5 32 Department of Orthodontics, School of Dentistry, University of Washington, Seattle, WA 33 34 35 98195 36 37 6 38 Department of Cell and Neurobiology, Keck School of Medicine, University of Southern 39 40 California, Los Angeles, CA 90033 41 42 43 Text pages: 28; Bibliography pages: 9; Figures: 6; Tables: 6 Appendices: 1 44 45 46 Running title: Babakotia radofilai postcranial suspensory adaptations 47 48 49 *Corresponding author: 50 51 52 Damiano Marchi 53 54 55 56 Address: Dipartimento di Biologia, Università di Pisa, Via Derna, 1 - ZIP 56126, Pisa - Italy 57 58 59 Ph: +39 050 2211350; Fax: +39 050 2211475 60 1 John Wiley & Sons Journal of Morphology Page 2 of 57 1 2 3 Email: [email protected] -
Reassessment of the Phylogenetic Relationships of the Late Miocene Apes Hispanopithecus and Rudapithecus Based on Vestibular Morphology
Reassessment of the phylogenetic relationships of the late Miocene apes Hispanopithecus and Rudapithecus based on vestibular morphology Alessandro Urciuolia,1, Clément Zanollib, Sergio Almécijaa,c,d, Amélie Beaudeta,e,f,g, Jean Dumoncelh, Naoki Morimotoi, Masato Nakatsukasai, Salvador Moyà-Solàa,j,k, David R. Begunl, and David M. Albaa,1 aInstitut Català de Paleontologia Miquel Crusafont, Universitat Autònoma de Barcelona, 08193 Barcelona, Spain; bUniv. Bordeaux, CNRS, MCC, PACEA, UMR 5199, F-33600 Pessac, France; cDivision of Anthropology, American Museum of Natural History, New York, NY 10024; dNew York Consortium in Evolutionary Primatology, New York, NY 10016; eDepartment of Archaeology, University of Cambridge, Cambridge CB2 1QH, United Kingdom; fSchool of Geography, Archaeology, and Environmental Studies, University of the Witwatersrand, Johannesburg, WITS 2050, South Africa; gDepartment of Anatomy, University of Pretoria, Pretoria 0001, South Africa; hLaboratoire Anthropology and Image Synthesis, UMR 5288 CNRS, Université de Toulouse, 31073 Toulouse, France; iLaboratory of Physical Anthropology, Graduate School of Science, Kyoto University, 606 8502 Kyoto, Japan; jInstitució Catalana de Recerca i Estudis Avançats, 08010 Barcelona, Spain; kUnitat d’Antropologia, Departament de Biologia Animal, Biologia Vegetal i Ecologia, Universitat Autònoma de Barcelona, 08193 Barcelona, Spain; and lDepartment of Anthropology, University of Toronto, Toronto, ON M5S 2S2, Canada Edited by Justin S. Sipla, University of Iowa, Iowa City, IA, and accepted by Editorial Board Member C. O. Lovejoy December 3, 2020 (received for review July 19, 2020) Late Miocene great apes are key to reconstructing the ancestral Dryopithecus and allied forms have long been debated. Until a morphotype from which earliest hominins evolved. Despite con- decade ago, several species of European apes from the middle sensus that the late Miocene dryopith great apes Hispanopithecus and late Miocene were included within this genus (9–16). -
Orangutan Positional Behavior and the Nature of Arboreal Locomotion in Hominoidea Susannah K.S
AMERICAN JOURNAL OF PHYSICAL ANTHROPOLOGY 000:000–000 (2006) Orangutan Positional Behavior and the Nature of Arboreal Locomotion in Hominoidea Susannah K.S. Thorpe1* and Robin H. Crompton2 1School of Biosciences, University of Birmingham, Edgbaston, Birmingham B15 2TT, UK 2Department of Human Anatomy and Cell Biology, University of Liverpool, Liverpool L69 3GE, UK KEY WORDS Pongo pygmaeus; posture; orthograde clamber; forelimb suspend ABSTRACT The Asian apes, more than any other, are and orthograde compressive locomotor modes are ob- restricted to an arboreal habitat. They are consequently an served more frequently. Given the complexity of orangu- important model in the interpretation of the morphological tan positional behavior demonstrated by this study, it is commonalities of the apes, which are locomotor features likely that differences in positional behavior between associated with arboreal living. This paper presents a de- studies reflect differences in the interplay between the tailed analysis of orangutan positional behavior for all age- complex array of variables, which were shown to influence sex categories and during a complete range of behavioral orangutan positional behavior (Thorpe and Crompton [2005] contexts, following standardized positional mode descrip- Am. J. Phys. Anthropol. 127:58–78). With the exception tions proposed by Hunt et al. ([1996] Primates 37:363–387). of pronograde suspensory posture and locomotion, orang- This paper shows that orangutan positional behavior is utan positional behavior is similar to that of the African highly complex, representing a diverse spectrum of posi- apes, and in particular, lowland gorillas. This study sug- tional modes. Overall, all orthograde and pronograde sus- gests that it is orthogrady in general, rather than fore- pensory postures are exhibited less frequently in the pres- limb suspend specifically, that characterizes the posi- ent study than previously reported. -
University of Illinois
UNIVERSITY OF ILLINOIS 3 MAY THIS IS TO CERTIFY THAT THE THESIS PREPARED UNDER MY SUPERVISION BY Leahanne M, Sarlo Functional Anatomy and Allometry in the Shoulder of ENTITLED........................................................ Suspensory Primates IS APPROVED BY ME AS FULFILLING THIS PART OF THE REQUIREMENTS FOR THE DEGREE OF. BACHELOR OF ARTS LIBERAL ARTS AND SCIENCES 0-1M4 t « w or c o w n w Introduction.....................................................................................................................................1 Dafinibon*..................................................................................................................................... 2 fFunctional m ivw w iiw irwbaola am foriwi irviiiiHbimanual iw 9 jrvafwooaitiona! Vf aai irwbahavlort iiHviw i# in thaahoukter joint complex......................................................................................3 nyvootiua pooioonaiaA^^AlttAAMAl oanainor................................................................................... i4 *4 -TnoOV a awmang.a Ia M AM JI* nwoo—U^AtkA^AA i (snDBDfllluu)/AuMyAlftAlAnAH |At AkJA^AAtlA•wwacwai* |A .............. 4ia i -Tho lar gibbon: KM obate* ]|£.................................................................................15 ANomatry.......................................................................................................................................16 Material* and matooda.....................................................................................................19 -
Functional Integration of the Hominin Forelimb by Marisa Elena Macias
Functional Integration of the Hominin Forelimb by Marisa Elena Macias Department of Evolutionary Anthropology Duke University Date:_______________________ Approved: ___________________________ Steven E. Churchill, Supervisor ___________________________ Katherine R. Saul ___________________________ Daniel O. Schmitt ___________________________ Christine E. Wall Dissertation submitted in partial fulfillment of the requirements for the degree of Doctor of Philosophy in the Department of Evolutionary Anthropology in the Graduate School of Duke University 2015 ABSTRACT Functional Integration of the Hominin Forelimb by Marisa Elena Macias Department of Evolutionary Anthropology Duke University Date:_______________________ Approved: ___________________________ Steven E. Churchill, Supervisor ___________________________ Katherine R. Saul ___________________________ Daniel O. Schmitt ___________________________ Christine E. Wall An abstract of a dissertation submitted in partial fulfillment of the requirements for the degree of Doctor of Philosophy in the Department of Evolutionary Anthropology in the Graduate School of Duke University 2015 Copyright by Marisa Elena Macias 2015 Abstract During the last six million years, humans shifted from a primarily arboreal lifestyle to a habitually bipedal, terrestrial lifestyle. Australopithecus had a significant bipedal component to its locomotion; whether suspensory and climbing behaviors were also important has remained unclear. Morphological features of the forelimb have been linked to locomotor differences among primates, but the interpretation of human fossils has remained problematic. This dissertation examines the total morphological pattern of the forelimb, specifically the functional integration of the musculature and joint systems. This approach employs both geometric morphometrics and a biomechanical modeling approach to study how and how well the forelimb morphology of living suspensory and quadrupedal primates, as well as humans and fossil hominins, accommodates climbing and suspensory locomotion. -
Cognitive Inferences in Fossil Apes (Primates, Hominoidea): Does Encephalization Reflect Intelligence?
JASs Invited Reviews Journal of Anthropological Sciences Vol. 88 (2010), pp. 11-48 Cognitive inferences in fossil apes (Primates, Hominoidea): does encephalization reflect intelligence? David M. Alba Institut Català de Paleontologia, Universitat Autònoma de Barcelona. Edifici ICP, Campus de la UAB s/n, 08193 Cerdanyola del Vallès, Barcelona (Spain); Dipartimento di Scienze della Terra, Università degli Studi di Firenze. Via G. La Pira 4, 50121 Firenze (Italy); e-mail: [email protected] Summary – Paleobiological inferences on general cognitive abilities (intelligence) in fossil hominoids strongly rely on relative brain size or encephalization, computed by means of allometric residuals, quotients or constants. This has been criticized on the basis that it presumably fails to reflect the higher intelligence of great apes, and absolute brain size has been favored instead. Many problems of encephalization metrics stem from the decrease of allometric slopes towards lower taxonomic level, thus making it difficult to determine at what level encephalization metrics have biological meaning. Here, the hypothesis that encephalization can be used as a good neuroanatomical proxy for intelligence is tested at two different taxonomic levels. A significant correlation is found between intelligence and encephalization only at a lower taxonomic level, i.e. on the basis of a low allometric slope, irrespective of whether species data or independent contrasts are employed. This indicates that higher-level slopes, resulting from encephalization grade shifts between subgroups (including hylobatids vs. great apes), do not reflect functional equivalence, whereas lower-level metrics can be employed as a paleobiological proxy for intelligence. Thus, in accordance to intelligence rankings, lower-level metrics indicate that great apes are more encephalized than both monkeys and hylobatids. -
Fleagle and Lieberman 2015F.Pdf
15 Major Transformations in the Evolution of Primate Locomotion John G. Fleagle* and Daniel E. Lieberman† Introduction Compared to other mammalian orders, Primates use an extraordinary diversity of locomotor behaviors, which are made possible by a complementary diversity of musculoskeletal adaptations. Primate locomotor repertoires include various kinds of suspension, bipedalism, leaping, and quadrupedalism using multiple pronograde and orthograde postures and employing numerous gaits such as walking, trotting, galloping, and brachiation. In addition to using different locomotor modes, pri- mates regularly climb, leap, run, swing, and more in extremely diverse ways. As one might expect, the expansion of the field of primatology in the 1960s stimulated efforts to make sense of this diversity by classifying the locomotor behavior of living primates and identifying major evolutionary trends in primate locomotion. The most notable and enduring of these efforts were by the British physician and comparative anatomist John Napier (e.g., Napier 1963, 1967b; Napier and Napier 1967; Napier and Walker 1967). Napier’s seminal 1967 paper, “Evolutionary Aspects of Primate Locomotion,” drew on the work of earlier comparative anatomists such as LeGros Clark, Wood Jones, Straus, and Washburn. By synthesizing the anatomy and behavior of extant primates with the primate fossil record, Napier argued that * Department of Anatomical Sciences, Health Sciences Center, Stony Brook University † Department of Human Evolutionary Biology, Harvard University 257 You are reading copyrighted material published by University of Chicago Press. Unauthorized posting, copying, or distributing of this work except as permitted under U.S. copyright law is illegal and injures the author and publisher. fig. 15.1 Trends in the evolution of primate locomotion. -
A New Hominoid-Bearing Locality from the Late Miocene of the Valles-Penedes Basin
Journal of Human Evolution xxx (2018) 1e11 Contents lists available at ScienceDirect Journal of Human Evolution journal homepage: www.elsevier.com/locate/jhevol Can Pallars i Llobateres: A new hominoid-bearing locality from the late Miocene of the Valles-Pened es Basin (NE Iberian Peninsula) * David M. Alba a, , Isaac Casanovas-Vilar a, Marc Furio a, b, Israel García-Paredes c, a, Chiara Angelone d, a, e, Sílvia Jovells-Vaque a, Angel H. Lujan f, a, g, Sergio Almecija h, a, 1, Salvador Moya-Sol a a, i, j a Institut Catala de Paleontologia Miquel Crusafont, Universitat Autonoma de Barcelona, Edifici ICTA-ICP, c/ Columnes s/n, Campus de la UAB, 08193 Cerdanyola del Valles, Barcelona, Spain b Departament de Geologia, Universitat Autonoma de Barcelona, 08193 Bellaterra, Spain c Departamento de Paleontología, Facultad de Ciencias Geologicas, Universidad Complutense de Madrid, c/ Jose Antonio Novais 2, 28040 Madrid, Spain d Dipartimento di Scienze, Universita Roma Tre, Largo San Leonardo Murialdo, 1, 00146, Roma, Italy e Institute of Vertebrate Paleontology and Paleoanthropology, Chinese Academy of Sciences, Xizhimen Wai Da Jie 142, Beijing 100044, China f Department of Geosciences, University of Fribourg, Chemin de Musee 6, 1700 Fribourg, Switzerland g Department of Geological Sciences, Faculty of Science, Masaryk University, Kotlarska 2, Brno, 611 37, Czech Republic h Center for the Advanced Study of Human Paleobiology, Department of Anthropology, The George Washington University, Washington, DC 20052, USA i Institucio Catalana de Recerca i Estudis Avançats (ICREA), Pg. Lluís Companys 23, 08010, Barcelona, Spain j Unitat d'Antropologia Biologica, Departament de Biologia Animal, Biologia Vegetal i Ecologia, Universitat Autonoma de Barcelona, 08193 Cerdanyola del Valles, Barcelona, Spain article info abstract Article history: In the Iberian Peninsula, Miocene apes (Hominoidea) are generally rare and mostly restricted to the Received 27 November 2017 Valles-Pened es Basin. -
The Evolution of Human and Ape Hand Proportions
ARTICLE Received 6 Feb 2015 | Accepted 4 Jun 2015 | Published 14 Jul 2015 DOI: 10.1038/ncomms8717 OPEN The evolution of human and ape hand proportions Sergio Alme´cija1,2,3, Jeroen B. Smaers4 & William L. Jungers2 Human hands are distinguished from apes by possessing longer thumbs relative to fingers. However, this simple ape-human dichotomy fails to provide an adequate framework for testing competing hypotheses of human evolution and for reconstructing the morphology of the last common ancestor (LCA) of humans and chimpanzees. We inspect human and ape hand-length proportions using phylogenetically informed morphometric analyses and test alternative models of evolution along the anthropoid tree of life, including fossils like the plesiomorphic ape Proconsul heseloni and the hominins Ardipithecus ramidus and Australopithecus sediba. Our results reveal high levels of hand disparity among modern hominoids, which are explained by different evolutionary processes: autapomorphic evolution in hylobatids (extreme digital and thumb elongation), convergent adaptation between chimpanzees and orangutans (digital elongation) and comparatively little change in gorillas and hominins. The human (and australopith) high thumb-to-digits ratio required little change since the LCA, and was acquired convergently with other highly dexterous anthropoids. 1 Center for the Advanced Study of Human Paleobiology, Department of Anthropology, The George Washington University, Washington, DC 20052, USA. 2 Department of Anatomical Sciences, Stony Brook University, Stony Brook, New York 11794, USA. 3 Institut Catala` de Paleontologia Miquel Crusafont (ICP), Universitat Auto`noma de Barcelona, Edifici Z (ICTA-ICP), campus de la UAB, c/ de les Columnes, s/n., 08193 Cerdanyola del Valle`s (Barcelona), Spain. -
Final Report on Environment Enhancement to Promote the Psychological Well-Being of Nonhuman Primates
Enviromental enhancement -- Non -Human Primates FINAL REPORT ON ENVIRONMENT ENHANCEMENT TO PROMOTE THE PSYCHOLOGICAL WELL-BEING OF NONHUMAN PRIMATES U. S. Department of Agriculture Animal and Plant Health Inspection Service Animal Care Riverdale, MD July 15, 1999 This Final Report contains the scientific basis for the Draft Policy and the methods we used in developing the Draft Policy. To view the Draft Policy and request for comments that was published in the Federal Register on July 15, 1999, click on Pdf TABLE OF CONTENTS I. INTRODUCTION AND PROJECT HISTORY A. Background on Evaluation of the Performance-Based Standard for Nonhuman Primates B. Team Methods C. Results of Surveys and Interviews II. PROMOTING PSYCHOLOGICAL WELL-BEING A. Intent and Language of the Animal Welfare Act B. Community Response C. Other Nations and Societies D. Difficulties Inherent in Measuring Psychological Well-being E. Species-Typical Behavior (STB) III. CRITICAL ELEMENT CONCEPT IV. LITERATURE REVIEW AND DISCUSSION A. Social Grouping B. Social Needs of Infants C. Structure and Substrate D. Foraging Opportunities E. Manipulanda F. Consideration of Sensory Stimulation G. Consideration of Novelty and Control V. REFERENCES AND OTHER SOURCES http://www.nal.usda.gov/awic/enrichment/Enviromental_Enhancement_NonHuman_Primates.htm[8/6/2015 1:02:44 PM] Enviromental enhancement -- Non -Human Primates A. References B. Other Sources APPENDIX A. 9 CFR Section 3. Environment Enhancement to Promote Psychological Well-Being of Nonhuman Primates APPENDIX B. Glossary APPENDIX C. Sample Species Information Sheets I. INTRODUCTION AND PROJECT HISTORY This report provides Animal and Plant Health Inspection Service (APHIS) Animal Care employees, the facilities they regulate, and the public with a policy on environment enhancement to promote the psychological well-being of nonhuman primates. -
Stable Isotope and Trace Element Paleoecology of the Rudabánya II Fauna: Paleoenvironmental Implications for the Late Miocene Hominoid, Rudapithecus Hungaricus
Stable Isotope and Trace Element Paleoecology of the Rudabánya II Fauna: Paleoenvironmental Implications for the Late Miocene Hominoid, Rudapithecus hungaricus by Laura Campbell Eastham A thesis submitted in conformity with the requirements for the degree of Doctor of Philosophy Graduate Department of Anthropology University of Toronto © Copyright by Laura C. Eastham 2017 Stable Isotope and Trace Element Paleoecology of the Rudabánya II Fauna: Paleoenvironmental Implications for the Late Miocene Hominoid, Rudapithecus hungaricus Laura C. Eastham Doctor of Philosophy Graduate Department of Anthropology University of Toronto 2017 Abstract The Late Miocene extinction of great apes in Europe has generally been regarded as the consequence of environmental changes that occurred in correlation with global Late Miocene cooling. However, given the range of dietary and locomotor adaptations observed among the different hominoid genera it is unlikely that the same environmental factors can account for the decline of the entire group. To better understand the factors that influenced the extinction of European Miocene apes it is necessary to evaluate their paleoecology on a regional scale. This research utilizes stable carbon and oxygen isotope (δ13C and δ18O) and strontium/calcium (Sr/Ca) trace element ratios measured in fossil ungulate tooth enamel to reconstruct the paleoecology and paleoclimate of Rudabánya II (R. II), an early Late Miocene (~10 Ma) hominoid locality in northeastern Hungary. The fossiliferous deposits at R. II preserve abundant samples of the extinct great ape Rudapithecus hungaricus. Primary aims of this research include: 1) evaluating the types of habitats present in terms of forest canopy cover, 2) examining trophic niche dynamics among the diverse ungulate community, and 3) estimating key climatic variables including mean annual temperature (MAT), mean annual precipitation (MAP), and degree of seasonality.