Copyright and Use of This Thesis This Thesis Must Be Used in Accordance with the Provisions of the Copyright Act 1968
Total Page:16
File Type:pdf, Size:1020Kb
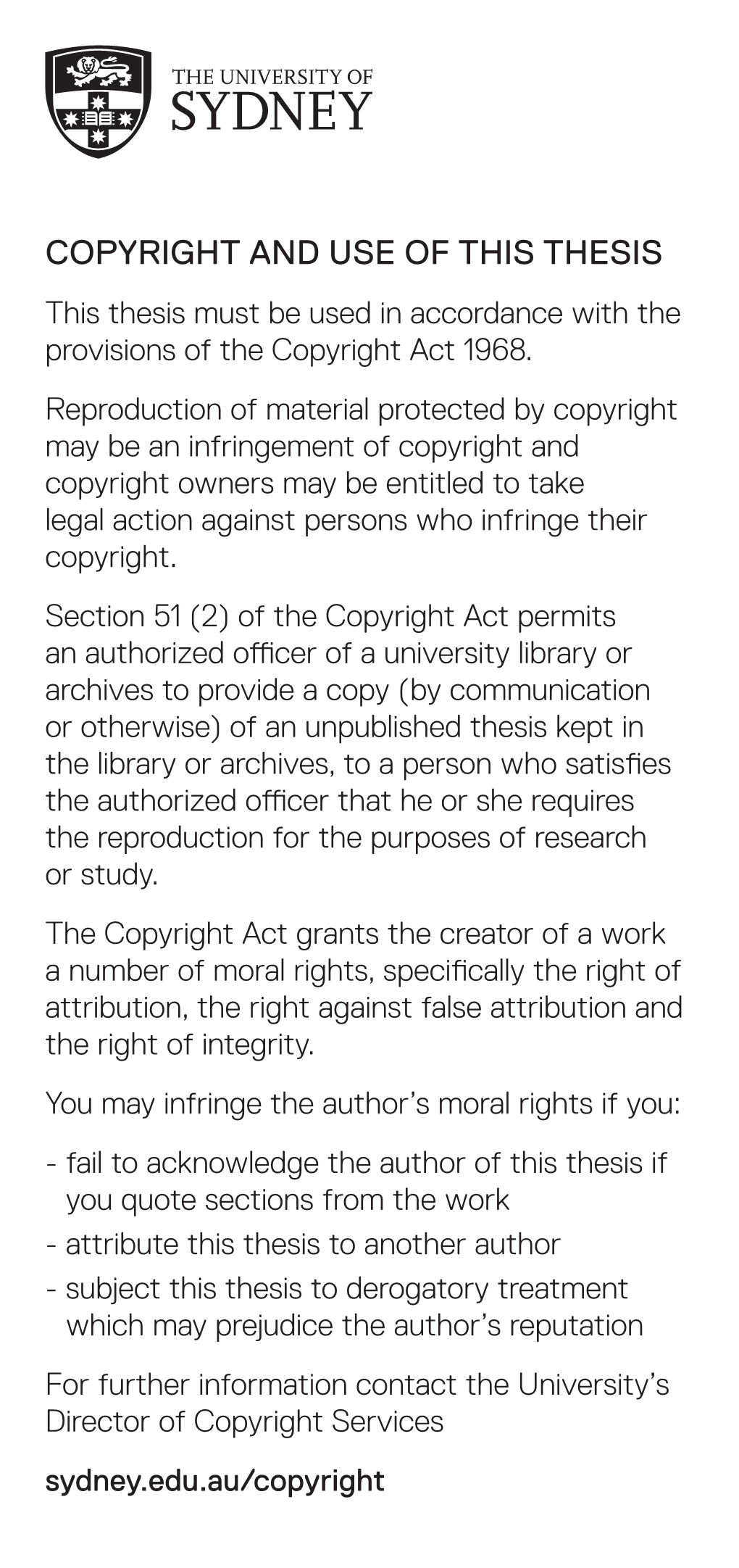
Load more
Recommended publications
-
Tiktaalik: Reconstructed at Left Tetrapod Testimony
Fa m i liar Fossils Tiktaalik fossil at right and Fossil site Tiktaalik: reconstructed at left Tetrapod Testimony IMAGINE a creature that looks like a crocodile with a neck but which also has gills like a fish! The stuff of nightmares, did you say? But it is just Tiktaalik roseae – a fish-like fossil that was introduced to the year old rocks. world on April 2006 by a team of researchers led by Neil They had a pretty Shubin, Edward Daeschler and Farish Jenkins, who clear idea about uncovered this bizarre specimen. It has been hailed as what sort of being the species that blurs the boundary between fish animal they were and the four-legged tetrapods. looking for. Tiktaalik was found on Ellesmere Island, Canada, However, success not too far from the North Pole in the Arctic Circle. It has was not been described as, “a cross between a fish and a crocodile.” immediate. It took It lived in the Devonian era lasting from 417m to 354m them five separate years ago. The name Tiktaalik is the Inuktitut word for expeditions to the freshwater fish burbot (Lota lota). The name was Canada before they suggested by Inuit elders of the area where the fossil was raised the discovered. The specific name roseae holds the clue to Tiktaalik. the name of an anonymous donor and is a tribute to the Tiktaalik was dubbed a “fishapod” by Daeschler. The person. media called it a “missing link” between fish and the Tiktaalik was a fish no doubt but a special one. Its four-legged tetrapods. -
On the Pelvic Girdle and Pin of Eusthenopteron. by Edwin S
PELVIC GIRDLE AND D'lN . OF EUSTHKNOPfERON. 311 On the Pelvic Girdle and Pin of Eusthenopteron. By Edwin S. Goodrich, M.A., Fellow of Mertoa College, Oxford. With Plate 16. 1 THROUGH the kindness of Mr. A. Smith Woodward, I have recently had the opportunity of looking through the fossil fish acquired by the British Museum since the Cata- logue was published. Amongst these was found a specimen of Eusthenopteron foordi, Whit., showing the endo- skeleton of the pelvic girdle and fin, of which I here give a description. The interest attaching to this fossil is con- siderable, since, of all the numerous extinct fish usually included in the group " Crossopterygii," it is the first and only one in which the parts of the skeleton of the pelvic girdle and its fin have been found complete and in their natural relations.2 The specimen (P. 6794) of which both the slab and the counterslab have been preserved, comes from the Upper Devonian of Canada. In it can be made out the skeleton of the pelvic girdle and fin of the right side, in a fairly com- plete and well-preserved condition, as represented in PI. 16, fig. 1, natural size. 1 To Mr. Smith Woodward I am also indebted for constant help when working in his Department. a The skeleton of the pelvic fin of Megalichthys has to some extent been made known by Cope, Miall, and Wellburn (2, 5, and 9), and the essential structure of that of Eusthenopteron has been briefly described by Traquair (7). VOL. 45, FART 2.—NEW SKKIES. -
AMS112 1978-1979 Lowres Web
--~--------~--------------------------------------------~~~~----------~-------------- - ~------------------------------ COVER: Paul Webber, technical officer in the Herpetology department searchers for reptiles and amphibians on a field trip for the Colo River Survey. Photo: John Fields!The Australian Museum. REPORT of THE AUSTRALIAN MUSEUM TRUST for the YEAR ENDED 30 JUNE , 1979 ST GOVERNMENT PRINTER, NEW SOUTH WALES-1980 D. WE ' G 70708K-1 CONTENTS Page Page Acknowledgements 4 Department of Palaeontology 36 The Australian Museum Trust 5 Department of Terrestrial Invertebrate Ecology 38 Lizard Island Research Station 5 Department of Vertebrate Ecology 38 Research Associates 6 Camden Haven Wildlife Refuge Study 39 Associates 6 Functional Anatomy Unit.. 40 National Photographic Index of Australian Director's Research Laboratory 40 Wildlife . 7 Materials Conservation Section 41 The Australian Museum Society 7 Education Section .. 47 Letter to the Premier 9 Exhibitions Department 52 Library 54 SCIENTIFIC DEPARTMENTS Photographic and Visual Aid Section 54 Department of Anthropology 13 PublicityJ Pu bl ications 55 Department of Arachnology 18 National Photographic Index of Australian Colo River Survey .. 19 Wildlife . 57 Lizard Island Research Station 59 Department of Entomology 20 The Australian Museum Society 61 Department of Herpetology 23 Appendix 1- Staff .. 62 Department of Ichthyology 24 Appendix 2-Donations 65 Department of Malacology 25 Appendix 3-Acknowledgements of Co- Department of Mammalogy 27 operation. 67 Department of Marine -
BOA2.1 Caecilian Biology and Natural History.Key
The Biology of Amphibians @ Agnes Scott College Mark Mandica Executive Director The Amphibian Foundation [email protected] 678 379 TOAD (8623) 2.1: Introduction to Caecilians Microcaecilia dermatophaga Synapomorphies of Lissamphibia There are more than 20 synapomorphies (shared characters) uniting the group Lissamphibia Synapomorphies of Lissamphibia Integumen is Glandular Synapomorphies of Lissamphibia Glandular Skin, with 2 main types of glands. Mucous Glands Aid in cutaneous respiration, reproduction, thermoregulation and defense. Granular Glands Secrete toxic and/or noxious compounds and aid in defense Synapomorphies of Lissamphibia Pedicellate Teeth crown (dentine, with enamel covering) gum line suture (fibrous connective tissue, where tooth can break off) basal element (dentine) Synapomorphies of Lissamphibia Sacral Vertebrae Sacral Vertebrae Connects pelvic girdle to The spine. Amphibians have no more than one sacral vertebrae (caecilians have none) Synapomorphies of Lissamphibia Amphicoelus Vertebrae Synapomorphies of Lissamphibia Opercular apparatus Unique to amphibians and Operculum part of the sound conducting mechanism Synapomorphies of Lissamphibia Fat Bodies Surrounding Gonads Fat Bodies Insulate gonads Evolution of Amphibians † † † † Actinopterygian Coelacanth, Tetrapodomorpha †Amniota *Gerobatrachus (Ray-fin Fishes) Lungfish (stem-tetrapods) (Reptiles, Mammals)Lepospondyls † (’frogomander’) Eocaecilia GymnophionaKaraurus Caudata Triadobatrachus Anura (including Apoda Urodela Prosalirus †) Salientia Batrachia Lissamphibia -
Cape Range National Park
Cape Range National Park Management Plan No 65 2010 R N V E M E O N G T E O H F T W A E I S L T A E R R N A U S T CAPE RANGE NATIONAL PARK Management Plan 2010 Department of Environment and Conservation Conservation Commission of Western Australia VISION By 2020, the park and the Ningaloo Marine Park will be formally recognised amongst the world’s most valuable conservation and nature based tourism icons. The conservation values of the park will be in better condition than at present. This will have been achieved by reducing stress on ecosystems to promote their natural resilience, and facilitating sustainable visitor use. In particular, those values that are not found or are uncommon elsewhere will have been conserved, and their special conservation significance will be recognised by the local community and visitors. The park will continue to support a wide range of nature-based recreational activities with a focus on preserving the remote and natural character of the region. Visitors will continue to enjoy the park, either as day visitors from Exmouth or by camping in the park itself at one of the high quality camping areas. The local community will identify with the park and the adjacent Ningaloo Marine Park, and recognise that its values are of international significance. An increasing number of community members will support and want to be involved in its ongoing management. The Indigenous heritage of the park will be preserved by the ongoing involvement of the traditional custodians, who will have a critical and active role in jointly managing the cultural and conservation values of the park. -
A New Osteolepidid Fish From
Rea. West. Aust. MU8. 1985, 12(3): 361-377 ANew Osteolepidid Fish from the Upper Devonian Gogo Formation, Western Australia J.A. Long* Abstract A new osteolepidid crossopterygian, Gogonasus andrewsi gen. et sp. nov., is des cribed from a single fronto-ethmoidal shield and associated ethmosphenoid, from the Late Devonian (Frasnian) Gogo Formation, Western Australia. Gogonasus is is distinguished from other osteolepids by the shape and proportions of the fronto ethmoidal shield, absence of palatal fenestrae, well developed basipterygoid pro cesses and moderately broad parasphenoid. The family Osteolepididae is found to be paraphyletic, with Gogonasus being regarded as a plesiomorphic osteolepidid at a similar level of organisation to Thursius. Introduction Much has been published on the well-preserved Late Devonian fish fauna from the Gogo Formation, Western Australia, although to date all the papers describing fish have been on placoderms (Miles 1971; Miles and Dennis 1979; Dennis and Miles 1979-1983; Young 1984), palaeoniscoids (Gardiner 1973, 1984; Gardiner and Bartram 1977) or dipnoans (Miles 1977; Campbell and Barwick 1982a, 1982b, 1983, 1984a). This paper describes the only osteolepiform from the fauna (Gardiner and Miles 1975), a small snout with associated braincase, ANU 21885, housed in the Geology Department, Australian National University. The specimen, collected by the Australian National University on the 1967 Gogo Expedition, was prepared by Dr S.M. Andrews (Royal Scottish Museum) and later returned to the ANU. Onychodus is the only other crossopterygian in the fauna. In its proportions and palatal structure the new specimen provides some additional new points of the anatomy of osteolepiforms. Few Devonian crossopte rygians are known from Australia, and so the specimen is significant in having resemblances to typical Northern Hemisphere species. -
Early Tetrapod Relationships Revisited
Biol. Rev. (2003), 78, pp. 251–345. f Cambridge Philosophical Society 251 DOI: 10.1017/S1464793102006103 Printed in the United Kingdom Early tetrapod relationships revisited MARCELLO RUTA1*, MICHAEL I. COATES1 and DONALD L. J. QUICKE2 1 The Department of Organismal Biology and Anatomy, The University of Chicago, 1027 East 57th Street, Chicago, IL 60637-1508, USA ([email protected]; [email protected]) 2 Department of Biology, Imperial College at Silwood Park, Ascot, Berkshire SL57PY, UK and Department of Entomology, The Natural History Museum, Cromwell Road, London SW75BD, UK ([email protected]) (Received 29 November 2001; revised 28 August 2002; accepted 2 September 2002) ABSTRACT In an attempt to investigate differences between the most widely discussed hypotheses of early tetrapod relation- ships, we assembled a new data matrix including 90 taxa coded for 319 cranial and postcranial characters. We have incorporated, where possible, original observations of numerous taxa spread throughout the major tetrapod clades. A stem-based (total-group) definition of Tetrapoda is preferred over apomorphy- and node-based (crown-group) definitions. This definition is operational, since it is based on a formal character analysis. A PAUP* search using a recently implemented version of the parsimony ratchet method yields 64 shortest trees. Differ- ences between these trees concern: (1) the internal relationships of aı¨stopods, the three selected species of which form a trichotomy; (2) the internal relationships of embolomeres, with Archeria -
<Article-Title>Your Inner Fish
ELIZABETHCOWLES, DEPARTMENT EDITOR We are happy to welcomeABM new Book ReviewsEditor, ElizabethCowles. ANIMAL EVOLUTION describeshis evolutionfrom "wetbehind the ears"novice to world famous,verte- R brate variousfield YourInner Fish: A Into the 3.5- paleontologist.Noting Journey he shares lessons Billion-Year of the Human experiences, important History Body. he has learned the He details Neil Shubin. 2008. Pantheon Books. along way. By from the to find- 240 Hardcover. everystep, planning trip (ISBN0375424474). pp. fossils to the $24.00. ing requiredpost-expedi- tion lab work. Of course, Shubin'smost In April2006, an above-the-foldstory impressive,logistically difficult expedition B on the front page of TheNew YorkTimes to date involvedfinding, unearthing, and introducedthe world to the fossilTiktaalik. extractingTiktaalik and thatreceives early, Composed of featuresthat are part fish prominenttreatment. Downloaded from http://online.ucpress.edu/abt/article-pdf/70/5/308/54744/30163281.pdf by guest on 29 September 2021 and Tiktaalikwas held part amphibian, But the real of this book is as an link"in ter- triumph up important"missing the ease with which Shubin the restrialvertebrate evolution. The fossiland plucks same in different its Dr. Neil were thus string many ways-name- discoverer, Shubin, that whatever thrust into the currentdebate ly, through organ system headlong or scientific lens it is on the of evolutionin the biol- analyzed viewed, teaching clearthat within fish areantecedents to all classroom.Shubin has on ogy capitalized limbed vertebrates.In discrete the fame the of chapters, accompanying discovery the evolution of terres- Tiktaalikto his firstbook YourInner Shubin explores pen trial ears,teeth, limbs, and chemore- Fish: A Journey Into the 3.5-Billion-Year eyes, ceptors from structureswithin fish (and History of the HumanBody. -
Center for Systematic Biology & Evolution
CENTER FOR SYSTEMATIC BIOLOGY & EVOLUTION 2008 ACTIVITY REPORT BY THE NUMBERS Research Visitors ....................... 253 Student Visitors.......................... 230 Other Visitors.......................... 1,596 TOTAL....................... 2,079 Outgoing Loans.......................... 535 Specimens/Lots Loaned........... 6,851 Information Requests .............. 1,294 FIELD WORK Botany - Uruguay Diatoms – Russia (Commander Islands, Kamchatka, Magdan) Entomology – Arizona, Colorado, Florida, Hawaii, Lesotho, Minnesota, Mississippi, Mongolia, Namibia, New Jersey, New Mexico, Ohio, Pennsylvania, South Africa, Tennessee LMSE – Zambia Ornithology – Alaska, England Vertebrate Paleontology – Canada (Nunavut Territory), Pennsylvania PROPOSALS BOTANY . Digitization of Latin American, African and other type specimens of plants at the Academy of Natural Sciences of Philadelphia, Global Plants Initiative (GPI), Mellon Foundation Award. DIATOMS . Algal Research and Ecologival Synthesis for the USGS National Water Quality Assessment (NAWQA) Program Cooperative Agreement 3. Co-PI with Don Charles (Patrick Center for Environmental Research, Phycology). Collaborative Research on Ecosystem Monitoring in the Russian Northern Far-East, Trust for Mutual Understanding Grant. CSBE Activity Report - 2008 . Diatoms of the Northcentral Pennsylvania, Pennsylvania Department of Conservation and Natural Resources, Wild Rescue Conservation Grant. Renovation and Computerization of the Diatom Herbarium at the Academy of Natural Sciences of Philadelphia, National -
Phylogeny of Basal Tetrapoda
Stuart S. Sumida Biology 342 Phylogeny of Basal Tetrapoda The group of bony fishes that gave rise to land-dwelling vertebrates and their descendants (Tetrapoda, or colloquially, “tetrapods”) was the lobe-finned fishes, or Sarcopterygii. Sarcoptrygii includes coelacanths (which retain one living form, Latimeria), lungfish, and crossopterygians. The transition from sarcopterygian fishes to stem tetrapods proceeded through a series of groups – not all of which are included here. There was no sharp and distinct transition, rather it was a continuum from very tetrapod-like fishes to very fish-like tetrapods. SARCOPTERYGII – THE LOBE-FINNED FISHES Includes •Actinista (including Coelacanths) •Dipnoi (lungfishes) •Crossopterygii Crossopterygians include “tetrapods” – 4- legged land-dwelling vertebrates. The Actinista date back to the Devonian. They have very well developed lobed-fins. There remains one livnig representative of the group, the coelacanth, Latimeria chalumnae. A lungfish The Crossopterygii include numerous representatives, the best known of which include Eusthenopteron (pictured here) and Panderichthyes. Panderichthyids were the most tetrapod-like of the sarcopterygian fishes. Panderichthyes – note the lack of dorsal fine, but retention of tail fin. Coelacanths Lungfish Rhizodontids Eusthenopteron Panderichthyes Tiktaalik Ventastega Acanthostega Ichthyostega Tulerpeton Whatcheeria Pederpes More advanced amphibians Tiktaalik roseae – a lobe-finned fish intermediate between typical sarcopterygians and basal tetrapods. Mid to Late Devonian; 375 million years old. The back end of Tiktaalik’s skull is intermediate between fishes and tetrapods. Tiktaalik is a fish with wrist bones, yet still retaining fin rays. The posture of Tiktaalik’s fin/limb is intermediate between that of fishes an tetrapods. Coelacanths Lungfish Rhizodontids Eusthenopteron Panderichthyes Tiktaalik Ventastega Acanthostega Ichthyostega Tulerpeton Whatcheeria Pederpes More advanced amphibians Reconstructions of the basal tetrapod Ventastega. -
Terrestrial-Style Feeding in a Very Early Aquatic Tetrapod Is Supported by Evidence from Experimental Analysis of Suture Morphology
Terrestrial-style feeding in a very early aquatic tetrapod is supported by evidence from experimental analysis of suture morphology Molly J. Markey*† and Charles R. Marshall*‡ *Department of Earth and Planetary Sciences and ‡Department of Organismic and Evolutionary Biology and Museum of Comparative Zoology, Harvard University, 26 Oxford Street, Cambridge, MA 02138 Communicated by Andrew H. Knoll, Harvard University, Cambridge, MA, March 6, 2007 (received for review September 25, 2006) There is no consensus on when in the fish-tetrapod transition stega, captured prey using suction or biting (15). Specifically, the suction feeding, the primary method of prey capture in the aquatic fishes Eusthenopteron, Panderichthys, and Tiktaalik, and the early realm, evolved into the direct biting on prey typical of terrestrial tetrapod Ventastega, all possess large coronoid fangs, whereas animals. Here, we show that differences in the morphology of se- these teeth are absent in the more derived Acanthostega.In lected cranial sutures between species that span the fish–tetrapod addition, Eusthenopteron and Panderichthys both exhibit an transition (the Devonian osteolepiform fish Eusthenopteron, the ossified operculum, whereas the bony gill cover is lost in aquatic Devonian tetrapod Acanthostega, and the Permian terres- Tiktaalik, Ventastega, and Acanthostega. Finally, the glenoid fossa trial tetrapod Phonerpeton) can be used to infer when terrestrial of the articular faces posteriordorsally in the fish taxa discussed feeding first appeared. Our approach consists of defining a sutural here (Eusthenopteron, Panderichthys, and Tiktaalik) whereas, in morphospace, assigning functional fields to that morphospace the tetrapods Ventastega and Acanthostega, this fossa points based on our previous measurements of suture function made dorsally, indicating that the lower jaw changed the nature of its during feeding in the living fish Polypterus, inferring the functions articulation to the skull across the fish–tetrapod transition. -
Teacher's Guide: Tiktaalik: a Fish out of Water
Teacher’s Guide: Tiktaalik: A Fish Out of Water Recommended Grade Level: 5–8 (also applicable to grades 9–12 for students requiring significant support in learning) Suggested Time: About 50–60 minutes spread over one or more class periods, plus additional time to complete a writing assignment Goals Vocabulary Following are the big ideas that students • fossil should take away after completing this lesson: • characteristic • Transitional fossils help scientists establish • transition how living things are related • tetrapod • Physical features and behaviors may • species change over time to help living things sur- vive where they live • amphibian • evolution Key Literacy Strategies Following are the primary literacy strategies students will use to complete this activity: • Categorizing basic facts and ideas (screen 10) • Making inferences (screens 4 and 7, writing assignment 2) • Identifying and using text features (screens 4, 6, and 9) • Determining important information (screen 7, writing assignment 1) • Sequencing events (screen 9) Note: In addition to using the key literacy strategies listed above, students will use each of the strategies below to complete this lesson: • Monitoring comprehension • Synthesizing • Making predictions • Developing vocabulary • Connecting prior knowledge to new learning • Developing a topic in writing • Identifying and using text features (photographs, captions, diagrams, and/or maps) Overview Tiktaalik: A Fish Out of Water is a student-directed learning experience. However, while students are expected to work through the lesson on their own, teachers should be available to keep the lesson on track, organize groupings, facilitate discussions, answer questions, and ensure that all learning goals are met. Teacher’s Guide: Tiktaalik: A Fish Out of Water 1 The following is a summary of the lesson screens: Screen 1: Students learn that they will explore evolutionary relationships between animal groups that do not appear to be related.