Supersymmetry at a 28 Tev Hadron Collider: HE-LHC
Total Page:16
File Type:pdf, Size:1020Kb
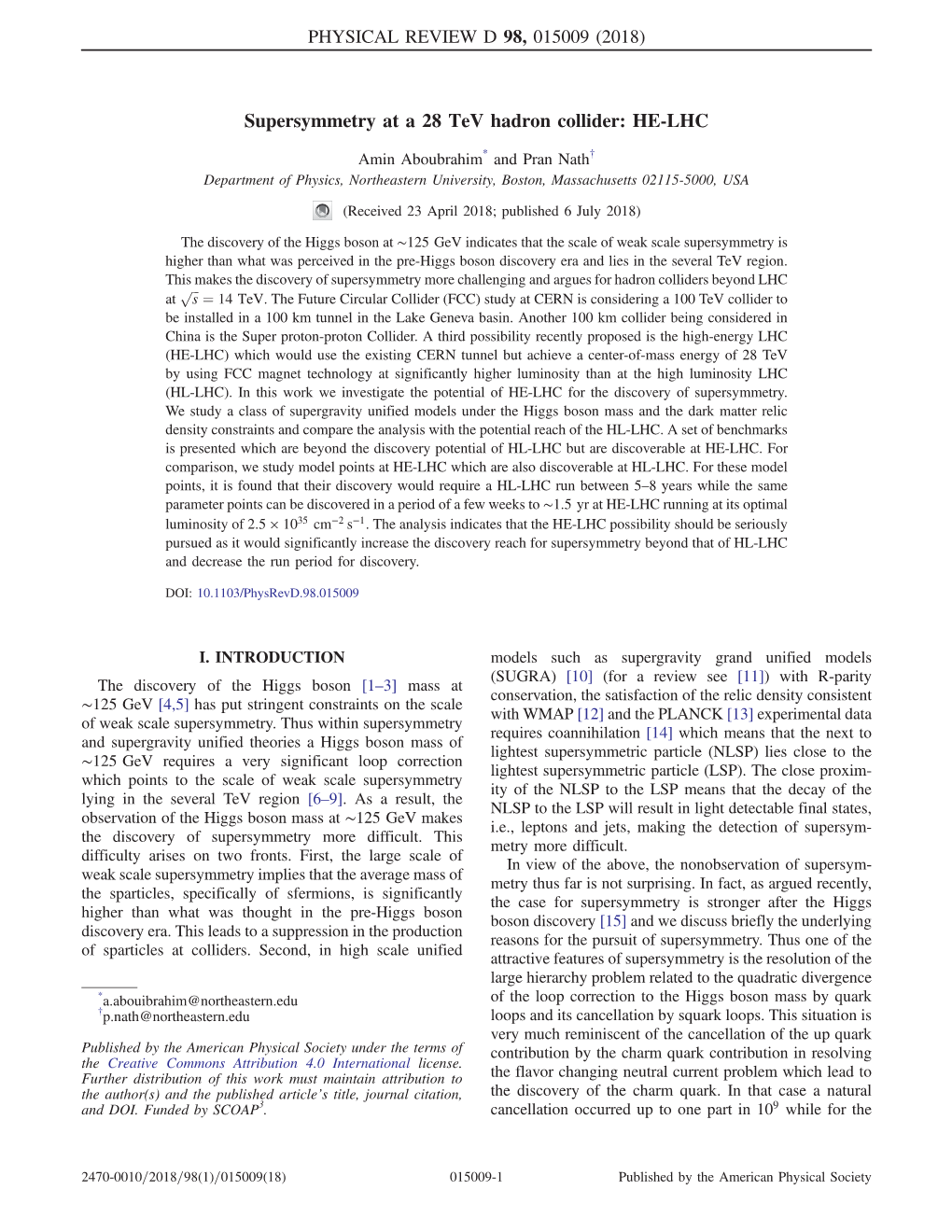
Load more
Recommended publications
-
Probing Supergravity Grand Unification in the Brookhaven G-2 Experiment
PHYSICAL REVIEW D VOLUME 53, NUMBER 3 1 FEBRUARY 1996 Probing supergravity grand unification in the Brookhaven 9 - 2 experiment Utpal Chattopadhyay Department of Physics, flovthe&tem University, Boston, Massachusetts 02115 Pran Nath Department of Physics, Northeastern University, Boston, Massachusetts 0211S and Institute for Theoretical Physics, University of California, Santa Barbara, California 93106-4030 (Received 24 July 1995) A quantitative analysis of a, 3 f(g - 2), within the framework of supergravity grand unification and radiative breaking of the electroweak symmetry is given. It is found that a;“sy is dominated by the chiial interference term from the light chargino exchange, and that this terni carries a signature which correlates strongly with the sign of p. Thus as a rule azusy > 0 for fl > 0 and I$“‘~ < 0 for ti < 0 with very few exceptions when tanp N 1. At the quantitative level it is shown that if the ES21 BNL experiment can reach the expected sensitivity of 4 x lo-” and there is a reduction in the hadronic error by a factor of 4 or more, then the experiment will explore a majority of the parameter space in the mo - mg plane in the region mo < 400 GeV, mg < 700 GeV for tanp > 10 assuming the experiment will not discard the standard model result within its 2~ uncertainty limit. For smaller tanp, the SUSY reach of ES21 will still be considerable. Further, if no effect within the 2~ limit of the standard model v&e is seen, then large tanp scenarios will he severely constrained within the current naturalness criterion, i.e , mo, mp < 1 TeV. -
Particles Meet Cosmology and Strings in Boston
PASCOS 2004 Particles meet cosmology and strings in Boston PASCOS 2004 is the latest in the symposium series that brings together disciplines from the frontier areas of modern physics. Participants at PASCOS 2004 and the Pran Nath Fest, which were held at Northeastern University, Boston. They include Howard Baer - front row sixth from left - then, moving right, Alfred Bartl, Michael Dine, Bruno Zumino, Pran Nath, Steven Weinberg, Paul Frampton, Mariano Quiros, Richard Arnowitt, MaryKGaillard, Peter Nilles and Michael Vaughn (chair, local organizing committee). The Tenth International Symposium on Particles, Strings and Cos redshift surveys suggests that the critical matter density of the uni mology took place at Northeastern University, Boston, on 16-22 verse is Qm ~ 0.3, direct dynamical measurements combined with August 2004. Two days of the symposium, 18-19 August, were the estimates of the luminosity density indicate Qm = 0.1-0.2. She devoted to the Pran Nath Fest in celebration of the 65th birthday of suggested that the apparent discrepancy may result from variations Matthews University Distinguished Professor Pran Nath. The PASCOS in the dark-matter fraction with mass and scale. She also suggested symposium is the largest interdisciplinary gathering on the interface that gravitational lensing maps combined with large redshift sur of the three disciplines of cosmology, particle physics and string veys promise to measure the dark-matter distribution in the uni theory, which have become increasingly entwined in recent years. verse. The microwave background can also provide clues to inflation Topics at PASCOS 2004 included the large-scale structure of the in the early universe. -
Susy 2018.Pdf
New ideas in Model Building Antonio Delgado University of Notre Dame SUSY2018 Barcelona, July 23-27, 2018 Internatonal Conference on Supersymmetry and Unification of Fundamental Interactons 2018 Internatonal Advisory Commitee Local Organising Commitee Ignatos Antoniadis, CERN Kaoru Hagiwara, KEK Martne Bosman, IFAE Lluïsa Mir, IFAE Csaba Balazs, Monash University Tao Han, Pitsburgh University Pilar Casado, UAB/IFAE Andrés Pacheco Pagés, IFAE/PIC Wim de Boer, KIT, Karlsruhe Gordon L. Kane, Michigan State University José Ramón Espinosa, ICREA/IFAE Alex Pomarol, UAB/IFAE Marcela Carena, Fermilab and Chicago University Dimitri Kazakov, JINR, Dubna Enrique Fernández, UAB/IFAE Oriol Pujolàs, IFAE Mirjam Cvetc, Pennsylvania State University Jihn E. Kim, Seoul Natonal University Sebastán Grinstein, ICREA/IFAE Mariano Quirós, IFAE, co-Chair Athanasios Dedes, Ioannina University Pyungwon Ko, KIAS, Seoul Aurelio Juste, ICREA/IFAE Javier Rico, IFAE Keith Dienes, Arizona State University Paul G. Langacker, IAS, Princeton Ilya Korolkov, IFAE Imma Riu, IFAE Herbi Dreiner, University of Bonn Joseph D. Lykken, Fermilab Mario Martnez, ICREA/IFAE, co-Chair Sebastán Grinschpun, IFAE John Ellis, King’s College UK & CERN Rabindra N. Mohapatra, University of Maryland Ramon Miquel, ICREA/IFAE Jonathan L. Feng, UC Irvine Pran Nath, Northeastern University Gian F. Giudice, CERN Apostolos Pilafsis, Manchester University Rohini M Godbole, CHEP, IISc, Bangalore Fernando Quevedo, ICTP Manoranjan Guchait, TIFR, Mumbai Graham G. Ross, University of Oxford John Gunion, UC Davis Sandip Trivedi, TIFR, Mumbai Pre-SUSY School July 17-20, 2018 susy2018.ifae.es Universitat Autònoma de Barcelona Organised by Supported by GOBIERNO MINISTERIO DE ESPAÑA DE ECONOMÍA, INDUSTRIA Y COMPETITIVIDAD Institut de Física d’Altes Energies Epicycles over epicycles Epicycles over epicycles Turtles over turtles • More seriously I am going to give my personal view on the status of Model Building. -
Probing Supergravity Unified Theories at the Large Hadron Collider
PROBING SUPERGRAVITY UNIFIED THEORIES AT THE LARGE HADRON COLLIDER A dissertation presented by Zuowei Liu to The Department of Physics In partial fulfilment of the requirements for the degree of Doctor of Philosophy in the field of arXiv:0808.3157v1 [hep-ph] 22 Aug 2008 Physics Northeastern University Boston, Massachusetts August, 2008 1 c Zuowei Liu, 2008 ALL RIGHTS RESERVED 2 PROBING SUPERGRAVITY UNIFIED THEORIES AT THE LARGE HADRON COLLIDER by Zuowei Liu ABSTRACT OF DISSERTATION Submitted in partial fulfillment of the requirement for the degree of Doctor of Philosophy in Physics in the Graduate School of Arts and Sciences of Northeastern University, August, 2008 3 Abstract The discovery of supersymmetry is one of the major goals of the current exper- iments at the Tevatron and in proposed experiments at the Large Hadron Collider (LHC). However when sparticles are produced the signatures of their production will to a significant degree depend on their hierarchical mass patterns. Here we investigate hierarchical mass patterns for the four lightest sparticles within one of the leading candidate theories - the SUGRA model. Specifically we analyze the hierarchies for the four lightest sparticles for the mSUGRA as well as for a general class of super- gravity unified models including nonuniversalities in the soft breaking sector. It is shown that out of nearly 104 possibilities of sparticle mass hierarchies, only a small number survives the rigorous constraints of radiative electroweak symmetry break- ing, relic density and other experimental constraints. The signature space of these mass patterns at the LHC is investigated using a large set of final states including multi-leptonic states, hadronically decaying τs, tagged b jets and other hadronic jets. -
Detecting Hidden Sector Dark Matter at HL-LHC and HE-LHC Via Long-Lived Stau Decays
PHYSICAL REVIEW D 99, 055037 (2019) Detecting hidden sector dark matter at HL-LHC and HE-LHC via long-lived stau decays † Amin Aboubrahim* and Pran Nath Department of Physics, Northeastern University, Boston, Massachusetts 02115-5000, USA (Received 14 February 2019; published 27 March 2019) We investigate a class of models where the supergravity model with the standard model gauge group is ð1Þ extended by a hidden sector U X gauge group and where the lightest supersymmetric particle is the neutralino in the hidden sector. We investigate this possibility in a class of models where the stau is the lightest supersymmetric particle in the minimal supersymmetric standard model sector and the next-to- ð1Þ lightest supersymmetric particle of the U X-extended supergravity model. In this case the stau will decay into the neutralino of the hidden sector. For the case when the mass gap between the stau and the hidden ð1Þ ð1Þ sector neutralino is small and the mixing between the U Y and U X is also small, the stau can decay into the hidden sector neutralino and a tau which may be reconstructed as a displaced track coming from a high-pT track of the charged stau. Simulations for this possibility are carried out for HL-LHC and HE-LHC. The discovery of such a displaced track from a stau will indicate the presence of hidden sector dark matter. DOI: 10.1103/PhysRevD.99.055037 I. INTRODUCTION highly suppressed couplings to the visible sector. Let us suppose that one of the two neutralinos which lie in the Most of the searches for dark matter (DM) are focused on hidden sector is the lightest supersymmetric particle (LSP) dark matter being a particle interacting weakly with the of the extended model and further the next-to-lightest standard model (SM) particles and having a cross section in supersymmetric particle (NLSP) is a stau which lies close a range accessible to direct detection and indirect detection to the hidden sector neutralino. -
PRAN NATH Department of Physics, Northeastern University Boston, MA 02115, USA
CALABI-YAU STRING THEORY-CURRENT STATUS PRAN NATH Department of Physics, Northeastern University Boston, MA 02115, USA and R. Arnowitt Center for Theoretical Physics, Department of Physics Texas A & M University, College Station, TX 77843 ABSTRACT Existence of 2 and probably more new light exotic states which are SU(3)cxSU(2)LxU(1) neutral is predicted within the framework of the three generation Calabi-Yau string models which flux break to [SU(3)J at the compactification scale, have intermediate scale breakdown to the Standard Model and possess matter parity invariance. It is shown that these exotic states mediate u eï and T -> uY decays with decay rates that may lie in the observable range. The final lepton is found to be right handed polarized. These phenomenon are predicted as experimental test of this class of string theories. Standard Model gauge group SU(3)cxSU(2) xU(1) I. INTRODUCTION L is accomplished by spontaneous breaking at an Calabi-Yau compactification of the intermediate scale Mi<Mc~MP£* Proton Heterotic String has been a subject of stability requires that the Calabi-Yau theory sonsiderable interest over the recent years below MQ be matter parity (M2) invariant [M =C U where C acts on the C-Y co-ordinates since it leads to physically interesting 2 z and obeys the condition C2=1 , while U is three gerneration models''*^. While it does z defined by diag U =(111)x(-1-11)x(-1-11). not seem likely that the three generation z Calabi-yau models as originally defined as Spontaneous breaking of [SU(3)]3 at Mj is corresponding to symmetric points in the accomplished by the VEV growth of an SO(10) c moduli space can be phenomenologically singlet field N and an SU(5) singlet field v viable, it appears that extensions of these belonging to the lepton nonet L(1,3,3). -
Reminiscences of My Work with Richard Lewis Arnowitt
Reminiscences of my work with Richard Lewis Arnowitt Pran Nath∗ Department of Physics, Northeastern University, Boston, MA 02115, USA This article contains reminiscences of the collaborative work that Richard Arnowitt and I did together which stretched over many years and encompasses several areas of particle theory. The article is an extended version of my talk at the Memorial Symposium in honor of Richard Arnowitt at Texas A&M, College Station, Texas, September 19-20, 2014. My collaboration with Richard Arnowitt (1928-2014)1 to constrain the parameters of the effective Lagrangian. started soon after I arrived at Northeastern University in The effective Lagrangian was a deduction from the fol- 1966 and continued for many years. In this brief article lowing set of conditions: (i) single particle saturation in on the reminiscences of my work with Dick I review some computation of T-products of currents, (ii) Lorentz in- of the more salient parts of our collaborative work which variance, (iii) “spectator” approximation, and (iv) local- includes effective Lagrangians, current algebra, scale in- ity which implies a smoothness assumption on the ver- variance and its breakdown, the U(1) problem, formu- tices. The above assumptions lead us to the conclusion lation of the first local supersymmetry and the develop- that the simplest way to achieve these constraints is via ment of supergravity grand unification in collaboration an effective Lagrangian which for T-products of three of Ali Chamseddine, and its applications to the search currents requires writing cubic interactions involving π,ρ for supersymmetry. and A1 fields and allowing for no derivatives in the first -order formalism and up to one-derivative in the second order formalism. -
AUB Physicist Ali Chamseddine Among TWAS Awardees
AUB Physicist Ali Chamseddine among TWAS awardees Friday October 30, 2009 The winners of the 2008 Academy of Sciences for the Developing World (TWAS) prizes in agricultural sciences, biology, chemistry, earth sciences, engineering sciences, mathematics, medical sciences, and physics were announced last month at the general meeting of TWAS held last month in Durban, South Africa. All the winners are from developing countries. The physics prize was won by AUB's Ali Hani Chamseddine and Predhiman Krishan Kaw, from India. Chamseddine was honored for his fundamental contributions to supergravity and noncommutative geometry. Born in Lebanon in 1953, Professor Chamseddine studied physics at the Lebanese University and was awarded a full scholarship to pursue graduate studies at Imperial College, London University, where he earned his PhD in 1976. He then did his postdoctoral study at the International Center for Theoretical Physics in Trieste, Italy before joining AUB as an assistant professor in 1977. Because of the war in Lebanon, in 1979 Chamseddine left Lebanon to work at the European organization for Nuclear Research in Switzerland; later, at Northeastern University in Boston, he produced ground- breaking research on particles physics with Richard Arnowitt and Pran Nath. Chamseddine returned to AUB in 1985, but then left again to work at the Swiss Federal Institute of Technology and at the University of Zurich. Returning to AUB in 1998, he became the founding director of the newly-established Center for Advanced Mathematical Sciences. Under his leadership, CAMS became the leading institute of mathematical sciences in the Middle East. He remained in this position until 2003 and now teaches in AUB's Physics Department. -
Researchers Pursue a Narrow Particle with Wide Implications 25 July 2006
Researchers Pursue a Narrow Particle with Wide Implications 25 July 2006 Northeastern University researchers Pran Nath, Gerlach Stueckelberg in 1938. While the Daniel Feldman and Zuowei Liu have shown that Stueckelberg mechanism arises naturally in string the discovery of a proposed particle, dubbed the theory, Kors and Nath were the first to successfully Stueckelberg Z prime, is possible utilizing the data utilize it in building a model of particle physics. being collected in the CDF and DO experiments at the Fermilab Tevatron. “If the Stueckelberg Z prime particle were to be discovered, it could signify a new kind of physics The Stueckelberg Z prime particle, originally altogether, a new regime so to speak,” said Nath. proposed by Boris Kors currently at CERN, “The prospect is quite exciting.” Geneva, Switzerland and Pran Nath at Northeastern University in 2004, is so narrow that Source: Northeastern University questions had been raised as to whether or not it could be detected. This new research, published in the July issue of Physical Review Letters, confirms that it can. The results are of importance because the discovery of this particle would provide a clue to the nature of physics beyond the Standard Model and a possible link with string theory. “It is exciting to know that the discovery of the proposed particle at colliders is indeed possible,” said Pran Nath, Matthews Distinguished University Professor of Physics at Northeastern University. “Physicists are always looking for what is next, what will lie beyond the Standard Model. These findings point us in the direction of those answers.” Because of its extreme narrowness, the Stueckelberg Z prime particle resembles the J/Psi (charmonium) particle, whose simultaneous discovery in 1974 by Burton Richter and Samuel Ting earned them the 1976 Nobel Prize in Physics. -
R-B in Supergravity Grand Unification with Nonuniversal Soft
PHYSICAL REVIEW D VOLUME 56, NUMBER 7 1 OCTOBER 1997 Rb in supergravity grand unification with nonuniversal soft supersymmetry breaking Tarakeshwar Dasgupta and Pran Nath Department of Physics, Northeastern University, Boston, Massachusetts 02115 ~Received 27 February 1997! An analysis of supersymmetric contributions to Rb in supergravity grand unification with nonuniversal boundary conditions on soft supersymmetry breaking in the scalar sector is given. Effects on Rb of Planck scale corrections on gaugino masses are also analyzed. It is found that there exist regions of the parameter space where positive corrections to Rb of size ;1s can be gotten. The region of the parameter space where enhancement of Rb occurs is identified. Predictions of sparticle masses for the maximal Rb case are given. The analysis has implications for the discovery of supersymmetric particles at colliders. @S0556-2821~97!00719-4# PACS number~s!: 12.60.Jv, 12.10.Dm, 13.38.Dg SM SUSY The standard model ~SM! predicts a value of Rb of Numerically Rb (0,0)50.2196 and ¹b (mt ,mb) is given by SM Rb 50.2159, mt5175 GeV ~1! SUSY a vLFL1vRFR ¹b ~mt ,mb!5 , ~4! with dRb /dmt520.0002. The experimental value of Rb has 2psin2u S ~v !21~v !2 D W L R been in a state of flux over the past couple of years. The experimental analyses in 1994–1995 indicated 1 1 2 expt where vL is defined by vL52 2 1 3 sin uW , and vR by Rb50.220860.0024. However, more recently Rb has 1 2 drifted downwards, and currently, assuming the value of Rc vR5 3 sin uW . -
Supersymmetry at a 28 Tev Hadron Collider: HE-LHC
Supersymmetry at a 28 TeV hadron collider: HE-LHC Amin Aboubrahim∗ and Pran Nathy Department of Physics, Northeastern University, Boston, MA 02115-5000, USA June 20, 2018 Abstract: The discovery of the Higgs boson at 125 GeV indicates that the scale of weak scale supersymmetry is higher than what was perceived∼ in the pre-Higgs boson discovery era and lies in the several TeV region. This makes the discovery of supersymmetry more challenging and argues for hadron colliders beyond LHC at ps = 14 TeV. The Future Circular Collider (FCC) study at CERN is considering a 100 TeV collider to be installed in a 100 km tunnel in the Lake Geneva basin. Another 100 km collider being considered in China is the Super proton-proton Collider (SppC). A third possibility recently proposed is the High-Energy LHC (HE-LHC) which would use the existing CERN tunnel but achieve a center-of-mass energy of 28 TeV by using FCC magnet technology at significantly higher luminosity than at the High Luminosity LHC (HL-LHC). In this work we investigate the potential of HE-LHC for the discovery of supersymmetry. We study a class of supergravity unified models under the Higgs boson mass and the dark matter relic density constraints and compare the analysis with the potential reach of the HL-LHC. A set of benchmarks are presented which are beyond the discovery potential of HL-LHC but are discoverable at HE-LHC. For comparison, we study model points at HE-LHC which are also discoverable at HL-LHC. For these model points, it is found that their discovery would require a HL-LHC run between 5-8 years while the same parameter points can be discovered in a period of few weeks to 1:5 yr at HE-LHC running at its optimal luminosity of 2:5 1035 cm−2 s−1. -
SUSY DARK MATTER Achille Corsetti and Pran Nath Department Of
View metadata, citation and similar papers at core.ac.uk brought to you by CORE provided by CERN Document Server SUSY DARK MATTER Achille Corsetti and Pran Nath Department of Physics, Northeastern University, Boston, MA 02115-5005, USA Abstract Several new effects have been investigated in recent analyses of supersymmetric dark matter. These include the effects of the uncertainties of wimp velocity dis- tributions, of the uncertainties of quark densities, of large CP violating phases, of nonuniversalities of the soft SUSY breaking parameters at the unification scale and of coannihilation on supersymmetric dark matter. We review here some of these with emphasis on the effects of nonuniversalities of the gaugino masses at the unification scale on the neutralino-proton cross-section from scalar interactions. The review encompasses several models where gaugino mass nonuniversalities occur including SUGRA models and D brane models. One finds that gaugino mass nonuniversalities can increase the scalar cross-sections by as much as a factor of 10 and also signifi- cantly extend the allowed range of the neutralino mass consistent with constraints up to about 500 GeV. These results have important implications for the search for supersymmetric dark matter. 1 INTRODUCTION Over the recent past there has been considerable experimental activity in the di- rect detection of dark matter 1, 2) and further progress is expected in the ongoing experiments 1, 2, 3) and new experiments that may come online in the future 4). At the same time there have been several theoretical developments which have shed light on the ambiguities and possible corrections that might be associated with the predictions on supersymmetric dark matter.