Tertiary Motifs in RNA Structure and Folding
Total Page:16
File Type:pdf, Size:1020Kb
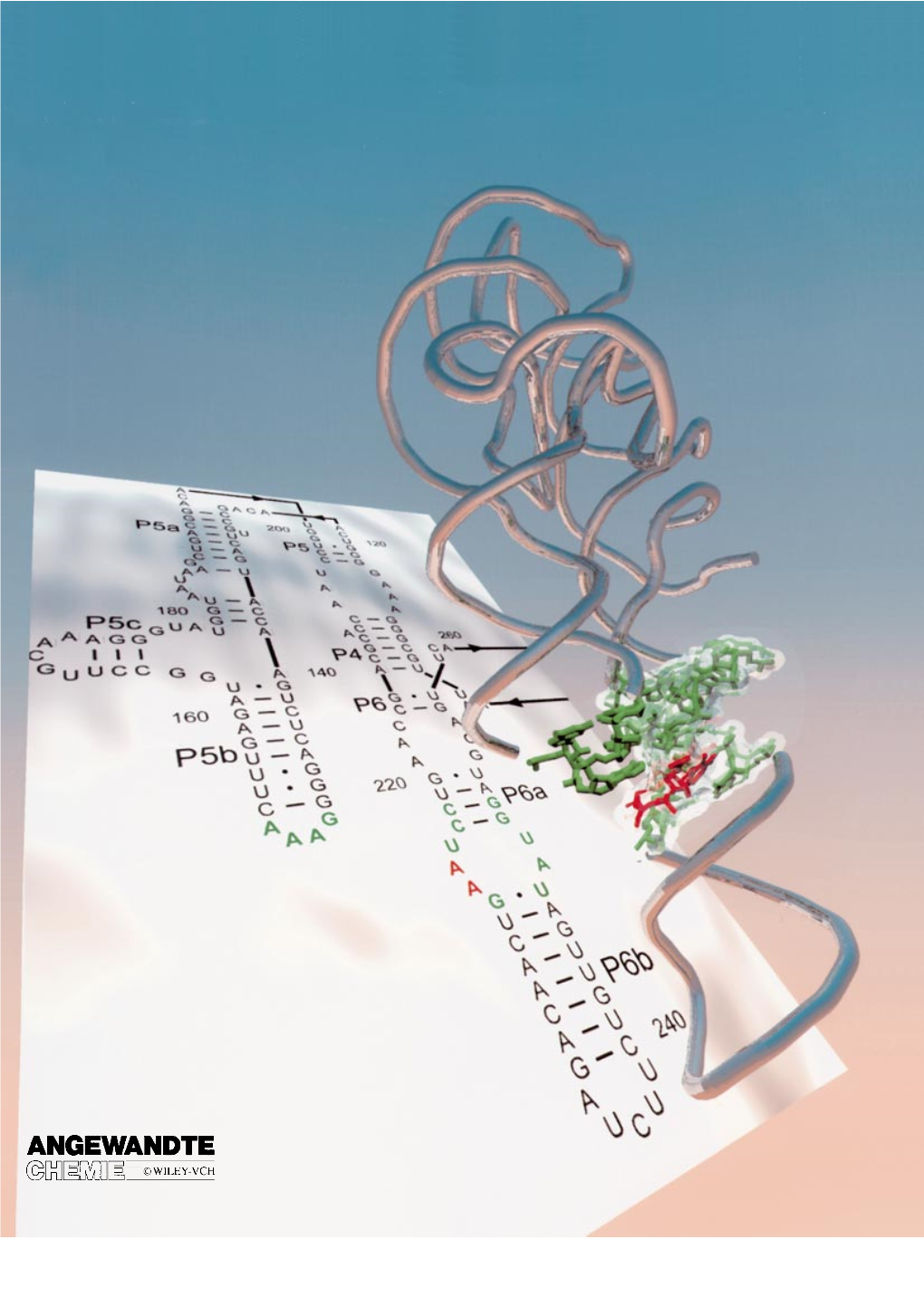
Load more
Recommended publications
-
Free-Energy Landscape of a Hyperstable RNA Tetraloop
Free-energy landscape of a hyperstable RNA tetraloop Jacob C. Minera,b, Alan A. Chenc, and Angel E. Garcíaa,d,1 aCenter for Nonlinear Studies, Los Alamos National Laboratory, Los Alamos, NM 87545; bDepartment of Biological Sciences, Rensselaer Polytechnic Institute, Troy, NY 12180; cDepartment of Chemistry, University at Albany, State University of New York, Albany, NY 12222; and dDepartment of Physics, Applied Physics, and Astronomy, Rensselaer Polytechnic Institute, Troy, NY 12180 Edited by Michael L. Klein, Temple University, Philadelphia, PA, and approved April 21, 2016 (received for review February 24, 2016) We report the characterization of the energy landscape and the through multiple configurational states (19, 21, 22, 28). This folding/unfolding thermodynamics of a hyperstable RNA tetraloop flexibility makes the GNRA tetraloop a useful target for ex- obtained through high-performance molecular dynamics simula- ploring both the accuracy of RNA force fields in recapitulating tions at microsecond timescales. Sampling of the configurational folded configurations and the heterogeneity of the folded free- landscape is conducted using temperature replica exchange molec- energy landscape. ular dynamics over three isochores at high, ambient, and negative In this report, we characterize the thermodynamics and folding pressures to determine the thermodynamic stability and the free- free-energy landscape for an oligonucleotide containing a GNRA energy landscape of the tetraloop. The simulations reveal reversible tetraloop sequence (r[gcGCAAgc]) through high-performance folding/unfolding transitions of the tetraloop into the canonical simulations and replica exchange molecular dynamics (REMD) A-RNA conformation and the presence of two alternative config- (29). The conformational ensembles are described in terms of urations, including a left-handed Z-RNA conformation and a compact global and local order parameters and reveal the formation of purine Triplet. -
Chapter 23 Nucleic Acids
7-9/99 Neuman Chapter 23 Chapter 23 Nucleic Acids from Organic Chemistry by Robert C. Neuman, Jr. Professor of Chemistry, emeritus University of California, Riverside [email protected] <http://web.chem.ucsb.edu/~neuman/orgchembyneuman/> Chapter Outline of the Book ************************************************************************************** I. Foundations 1. Organic Molecules and Chemical Bonding 2. Alkanes and Cycloalkanes 3. Haloalkanes, Alcohols, Ethers, and Amines 4. Stereochemistry 5. Organic Spectrometry II. Reactions, Mechanisms, Multiple Bonds 6. Organic Reactions *(Not yet Posted) 7. Reactions of Haloalkanes, Alcohols, and Amines. Nucleophilic Substitution 8. Alkenes and Alkynes 9. Formation of Alkenes and Alkynes. Elimination Reactions 10. Alkenes and Alkynes. Addition Reactions 11. Free Radical Addition and Substitution Reactions III. Conjugation, Electronic Effects, Carbonyl Groups 12. Conjugated and Aromatic Molecules 13. Carbonyl Compounds. Ketones, Aldehydes, and Carboxylic Acids 14. Substituent Effects 15. Carbonyl Compounds. Esters, Amides, and Related Molecules IV. Carbonyl and Pericyclic Reactions and Mechanisms 16. Carbonyl Compounds. Addition and Substitution Reactions 17. Oxidation and Reduction Reactions 18. Reactions of Enolate Ions and Enols 19. Cyclization and Pericyclic Reactions *(Not yet Posted) V. Bioorganic Compounds 20. Carbohydrates 21. Lipids 22. Peptides, Proteins, and α−Amino Acids 23. Nucleic Acids ************************************************************************************** -
Structure and Thermodynamics of a Conserved U2 Snrna Domain from Yeast and Human
JOBNAME: RNA 13#3 2007 PAGE: 1 OUTPUT: Wednesday January 17 20:23:55 2007 csh/RNA/131630/rna4184 Downloaded from rnajournal.cshlp.org on September 29, 2021 - Published by Cold Spring Harbor Laboratory Press Structure and thermodynamics of a conserved U2 snRNA domain from yeast and human DIPALI G. SASHITAL,1 VINCENZO VENDITTI,2 CORTNEY G. ANGERS,1,4 GABRIEL CORNILESCU,3 and SAMUEL E. BUTCHER1 1Department of Biochemistry, University of Wisconsin–Madison, Madison, Wisconsin 53706, USA 2Biomolecular Structure Research Center and Dipartimento di Biologia Molecolare, Universita di Siena, I-53100 Siena, Italy 3National Magnetic Resonance Facility at Madison, Department of Biochemistry, University of Wisconsin–Madison, Madison, Wisconsin 53706, USA ABSTRACT The spliceosome is a dynamic ribonucleoprotein complex responsible for the removal of intron sequences from pre-messenger RNA. The highly conserved 59 end of the U2 small nuclear RNA (snRNA) makes key base-pairing interactions with the intron branch point sequence and U6 snRNA. U2 stem I, a stem–loop located in the 59 region of U2, has been implicated in spliceosome assembly and may modulate the folding of the U2 and U6 snRNAs in the spliceosome active site. Here we present the NMR structures of U2 stem I from human and Saccharomyces cerevisiae. These sequences represent the two major classes of U2 stem I, distinguished by the identity of tandem wobble pairs (UU/UU in yeast and CA/GU in human) and the presence of post-transcriptional modifications (four 29-O-methyl groups and two pseudouracils in human). The structures reveal that the UU/UU and CA/GU tandem wobble pairs are nearly isosteric. -
1 History for Canonical and Non-Canonical Structures of Nucleic Acids
1 1 History for Canonical and Non-canonical Structures of Nucleic Acids The main points of the learning: Understand canonical and non-canonical structures of nucleic acids and think of historical scientists in the research field of nucleic acids. 1.1 Introduction This book is to interpret the non-canonical structures and their stabilities of nucleic acids from the viewpoint of the chemistry and study their biological significances. There is more than 60 years’ history after the discovery of the double helix DNA structure by James Dewey Watson and Francis Harry Compton Crick in 1953, and chemical biology of nucleic acids is facing a new aspect today. Through this book, I expect that readers understand how the uncommon structure of nucleic acids became one of the common structures that fascinate us now. In this chapter, I introduce the history of nucleic acid structures and the perspective of research for non-canonical nucleic acid structures (see also Chapter 15). 1.2 History of Duplex The opening of the history of genetics was mainly done by three researchers. Charles Robert Darwin, who was a scientist of natural science, pioneered genetics. The proposition of genetic concept is indicated in his book On the Origin of Species published in 1859. He indicated the theory of biological evolution, which is the basic scientific hypothesis of natural diversity. In other words, he proposed biological evolution, which changed among individuals by adapting to the environment and be passed on to the next generation. However, that was still a primitive idea for the genetic concept. After that, Gregor Johann Mendel, who was a priest in Brno, Czech Republic, confirmed the mechanism of gene evolution by using “factor” inherited from parent to children using pea plant in 1865. -
Accurate Energies of Hydrogen Bonded Nucleic Acid Base Pairs and Triplets in Trna Tertiary Interactions Romina Oliva1,2,*, Luigi Cavallo3 and Anna Tramontano2
View metadata, citation and similar papers at core.ac.uk brought to you by CORE provided by PubMed Central Nucleic Acids Research, 2006, Vol. 34, No. 3 865–879 doi:10.1093/nar/gkj491 Accurate energies of hydrogen bonded nucleic acid base pairs and triplets in tRNA tertiary interactions Romina Oliva1,2,*, Luigi Cavallo3 and Anna Tramontano2 1Centro Linceo Interdisciplinare ‘Beniamino Segre’, Accademia dei Lincei, I-00165 Rome, Italy, 2Dipartimento di Scienze Biochimiche ‘A. Rossi Fanelli’, Universita` di Roma ‘La Sapienza’, I-00185 Rome, Italy and 3Dipartimento di Chimica, Universita` di Salerno, Via Salvador Allende, Baronissi (SA), I-84081, Italy Received December 4, 2005; Revised and Accepted January 19, 2006 ABSTRACT intercalated A58–G18–A/G57–G19 purine bases. All these interactions are highly conserved in cytosolic tRNAs, indic- Tertiary interactions are crucial in maintaining the ating their importance for the tRNA function. Available tRNA structure and functionality. We used a com- experimental data also support the functional importance of bined sequence analysis and quantum mechanics such a region. Mutants of the Escherichia coli tRNAAla(CUA) approach to calculate accurate energies of the most obtained by random mutagenesis and having at least one of frequent tRNA tertiary base pairing interactions. Our the above-mentioned interactions disrupted were shown to be analysis indicates that six out of the nine classical non-functional (6), and insertion of additional nucleotides into tertiary interactions are held in place mainly by the T-loop or deletion of G19 from the D-loop affected the H-bonds between the bases. In the remaining three accuracy of the codon–anticodon recognition, resulting in a cases other effects have to be considered. -
An O(N5) Algorithm for MFE Prediction of Kissing Hairpins and 4-Chains in Nucleic Acids
JOURNAL OF COMPUTATIONAL BIOLOGY Volume 16, Number 6, 2009 # Mary Ann Liebert, Inc. Pp. 803–815 DOI: 10.1089/cmb.2008.0219 An O(n5) Algorithm for MFE Prediction of Kissing Hairpins and 4-Chains in Nucleic Acids HO-LIN CHEN,1 ANNE CONDON,2 and HOSNA JABBARI2 ABSTRACT Efficient methods for prediction of minimum free energy (MFE) nucleic secondary struc- tures are widely used, both to better understand structure and function of biological RNAs and to design novel nano-structures. Here, we present a new algorithm for MFE secondary structure prediction, which significantly expands the class of structures that can be handled in O(n5) time. Our algorithm can handle H-type pseudoknotted structures, kissing hairpins, and chains of four overlapping stems, as well as nested substructures of these types. Key words: kissing hairpins, pseudoknot, RNA, secondary structure prediction. 1. INTRODUCTION ur knowledge of the amazing variety of functions played by RNA molecules in the cell Ocontinues to expand, with the functions determined in part by structure (Lee et al., 1997). Additionally, DNA and RNA sequences are designed to form novel structures for a wide range of applications, such as algorithmic DNA self-assembly (He et al., 2008; Rothemund et al., 2004), detection of low concentrations of other molecules of interest (Dirks and Pierce, 2004) or to exhibit motion (Simmel and Dittmer, 2005). In order to improve our ability to determine function from DNA or RNA sequences, and also to aid in the design of nucleic acids with novel structural or functional properties, accurate and efficient methods for predicting nucleic acid structure are very valuable. -
Nucleotides and Nucleic Acids
Nucleotides and Nucleic Acids Energy Currency in Metabolic Transactions Essential Chemical Links in Response of Cells to Hormones and Extracellular Stimuli Nucleotides Structural Component Some Enzyme Cofactors and Metabolic Intermediate Constituents of Nucleic Acids: DNA & RNA Basics about Nucleotides 1. Term Gene: A segment of a DNA molecule that contains the information required for the synthesis of a functional biological product, whether protein or RNA, is referred to as a gene. Nucleotides: Nucleotides have three characteristic components: (1) a nitrogenous (nitrogen-containing) base, (2) a pentose, and (3) a phosphate. The molecule without the phosphate groups is called a nucleoside. Oligonucleotide: A short nucleic acid is referred to as an oligonucleotide, usually contains 50 or fewer nucleotides. Polynucleotide: Polymers containing more than 50 nucleotides is usually referred to as polynucleotide. General structure of nucleotide, including a phosphate group, a pentose and a base unit (either Purine or Pyrimidine). Major purine and Pyrimidine bases of nucleic acid The roles of RNA and DNA DNA: a) Biological Information Storage, b) Biological Information Transmission RNA: a) Structural components of ribosomes and carry out the synthesis of proteins (Ribosomal RNAs: rRNA); b) Intermediaries, carry genetic information from gene to ribosomes (Messenger RNAs: mRNA); c) Adapter molecules that translate the information in mRNA to proteins (Transfer RNAs: tRNA); and a variety of RNAs with other special functions. 1 Both DNA and RNA contain two major purine bases, adenine (A) and guanine (G), and two major pyrimidines. In both DNA and RNA, one of the Pyrimidine is cytosine (C), but the second major pyrimidine is thymine (T) in DNA and uracil (U) in RNA. -
The Structure and Function of Large Biological Molecules 5
The Structure and Function of Large Biological Molecules 5 Figure 5.1 Why is the structure of a protein important for its function? KEY CONCEPTS The Molecules of Life Given the rich complexity of life on Earth, it might surprise you that the most 5.1 Macromolecules are polymers, built from monomers important large molecules found in all living things—from bacteria to elephants— can be sorted into just four main classes: carbohydrates, lipids, proteins, and nucleic 5.2 Carbohydrates serve as fuel acids. On the molecular scale, members of three of these classes—carbohydrates, and building material proteins, and nucleic acids—are huge and are therefore called macromolecules. 5.3 Lipids are a diverse group of For example, a protein may consist of thousands of atoms that form a molecular hydrophobic molecules colossus with a mass well over 100,000 daltons. Considering the size and complexity 5.4 Proteins include a diversity of of macromolecules, it is noteworthy that biochemists have determined the detailed structures, resulting in a wide structure of so many of them. The image in Figure 5.1 is a molecular model of a range of functions protein called alcohol dehydrogenase, which breaks down alcohol in the body. 5.5 Nucleic acids store, transmit, The architecture of a large biological molecule plays an essential role in its and help express hereditary function. Like water and simple organic molecules, large biological molecules information exhibit unique emergent properties arising from the orderly arrangement of their 5.6 Genomics and proteomics have atoms. In this chapter, we’ll first consider how macromolecules are built. -
Chapter 22. Nucleic Acids
Chapter 22. Nucleic Acids 22.1 Types of Nucleic Acids 22.2 Nucleotides: Building Blocks of Nucleic Acids 22.3 Primary Nucleic Acid Structure 22.4 The DNA Double Helix 22.5 Replication of DNA Molecules 22.6 Overview of Protein Synthesis 22.7 Ribonucleic Acids Chemistry at a Glance: DNA Replication 22.8 Transcription: RNA Synthesis 22.9 The Genetic Code 22.10 Anticodons and tRNA Molecules 22.11 Translation: Protein Synthesis 22.12 Mutations Chemistry at a Glance: Protein Synthesis 22.13 Nucleic Acids and Viruses 22.14 Recombinant DNA and Genetic Engineering 22.15 The Polymerase Chain Reaction 22.16 DNA Sequencing Students should be able to: 1. Relate DNA to genes and chromosomes. 2. Describe the structure of a molecule of DNA including the base-pairing pattern. 3. Describe the structure of a nucleotide of RNA. 4. Describe the structure of a molecule of RNA. 5. Describe the three kinds of RNA and construct a pictorial representation. 6. Summarize the physiology of DNA in terms of replication and protein synthesis. 7. List the sequence of events in DNA replication and explain why it is referred to as semiconservative. 8. Evaluate the process of transcription. 9. Evaluate the process of translation. 10. Given a DNA coding strand and the genetic code , determine the complementary messenger RNA strand, the codons that would be involved in peptide formation from the messenger RNA sequence, and the amino acid sequence that would be translated. 11. Define mutation. 12. Differentiate between base substitutions and base insertions and/or deletions. 13. Discuss sickle-cell anemia. -
Plant G-Quadruplex (G4) Motifs in DNA and RNA; Abundant, Intriguing Sequences of Unknown Function T
Plant Science 269 (2018) 143–147 Contents lists available at ScienceDirect Plant Science journal homepage: www.elsevier.com/locate/plantsci Review article Review: Plant G-quadruplex (G4) motifs in DNA and RNA; abundant, intriguing sequences of unknown function T ⁎ Brianna D. Griffin, Hank W. Bass Department of Biological Science, 319 Stadium Drive, Florida State University, Tallahassee, FL, 32306-4295, USA ARTICLE INFO ABSTRACT Keywords: DNA sequences capable of forming G-quadruplex (G4) structures can be predicted and mapped in plant genomes G-quadruplex using computerized pattern search programs. Non-telomeric G4 motifs have recently been found to number in G4 the thousands across many plant species and enriched around gene promoters, prompting speculation that they Gene regulation may represent a newly uncovered and ubiquitous family of cis-acting elements. Comparative analysis shows that cis-regulatory element monocots exhibit five to ten times higher G4 motif density than eudicots, but the significance of this difference has not been determined. The vast scale and complexity of G4 functions, actual or theoretical, are reviewed in relation to the multiple modes of action and myriad genetic functions for which G4s have been implicated in DNA and RNA. Future experimental strategies and opportunities include identifying plant G4-interactomes, resolving the structures of G4s with and without their binding partners, and defining molecular mechanisms through reporter gene, genetic, or genome editing approaches. Given the global importance of plants for food, clothing, medicine, and energy, together with the potential role of G4 motifs as a widely conserved set of DNA sequences that could coordinate gene regulation, future plant G4 research holds great potential for use in plant improvement strategies. -
De Novo Nucleic Acids: a Review of Synthetic Alternatives to DNA and RNA That Could Act As † Bio-Information Storage Molecules
life Review De Novo Nucleic Acids: A Review of Synthetic Alternatives to DNA and RNA That Could Act as y Bio-Information Storage Molecules Kevin G Devine 1 and Sohan Jheeta 2,* 1 School of Human Sciences, London Metropolitan University, 166-220 Holloway Rd, London N7 8BD, UK; [email protected] 2 Network of Researchers on the Chemical Evolution of Life (NoR CEL), Leeds LS7 3RB, UK * Correspondence: [email protected] This paper is dedicated to Professor Colin B Reese, Daniell Professor of Chemistry, Kings College London, y on the occasion of his 90th Birthday. Received: 17 November 2020; Accepted: 9 December 2020; Published: 11 December 2020 Abstract: Modern terran life uses several essential biopolymers like nucleic acids, proteins and polysaccharides. The nucleic acids, DNA and RNA are arguably life’s most important, acting as the stores and translators of genetic information contained in their base sequences, which ultimately manifest themselves in the amino acid sequences of proteins. But just what is it about their structures; an aromatic heterocyclic base appended to a (five-atom ring) sugar-phosphate backbone that enables them to carry out these functions with such high fidelity? In the past three decades, leading chemists have created in their laboratories synthetic analogues of nucleic acids which differ from their natural counterparts in three key areas as follows: (a) replacement of the phosphate moiety with an uncharged analogue, (b) replacement of the pentose sugars ribose and deoxyribose with alternative acyclic, pentose and hexose derivatives and, finally, (c) replacement of the two heterocyclic base pairs adenine/thymine and guanine/cytosine with non-standard analogues that obey the Watson–Crick pairing rules. -
RNA Tertiary Structure Energetics Predicted by an Ensemble Model of the RNA Double Helix
bioRxiv preprint doi: https://doi.org/10.1101/341107; this version posted June 6, 2018. The copyright holder for this preprint (which was not certified by peer review) is the author/funder. All rights reserved. No reuse allowed without permission. TITLE RNA tertiary structure energetics predicted by an ensemble model of the RNA double helix 1 Joseph D. Yesselman * 2 Sarah K. Denny * Namita Bisaria1,† Daniel Herschlag1,5,6,‡ William J. Greenleaf2,3,4,5,7,‡ Rhiju Das1,8,‡ 1 Department of Biochemistry, Stanford University, Stanford, CA 94305, United States 2 Program in Biophysics, Stanford University, Stanford, CA 94305, United States 3 Department of Genetics, Stanford University, Stanford, CA 94305, United States 4 Department of Applied Physics, Stanford University, Stanford, CA 94305, United States 5 Department of Chemistry, Stanford University, Stanford, CA 94305, United States 6 Stanford ChEM-H (Chemistry, Engineering, and Medicine for Human Health), Stanford University, Stanford, California 94305, USA 7 Chan Zuckerberg Biohub, San Francisco, CA, United States 8 Department of Physics, Stanford University, Stanford, CA 94305, United States *These authors contributed equally † Current address: Whitehead Institute for Biomedical Research, Cambridge, MA 02142, United States ‡ Correspondence should be addressed to D.H. ([email protected]), W.J.G ([email protected]), or R.D. ([email protected]). Classification: Biological Sciences – Biophysics Keywords: Blind Prediction, RNA Energetics, High-throughput Data Short title: Blind Predictions of RNA Tertiary Assembly Energetics 1 bioRxiv preprint doi: https://doi.org/10.1101/341107; this version posted June 6, 2018. The copyright holder for this preprint (which was not certified by peer review) is the author/funder.