A Theoretical Investigation of Convective Patterns in the Lee of the Colorado Rockies
Total Page:16
File Type:pdf, Size:1020Kb
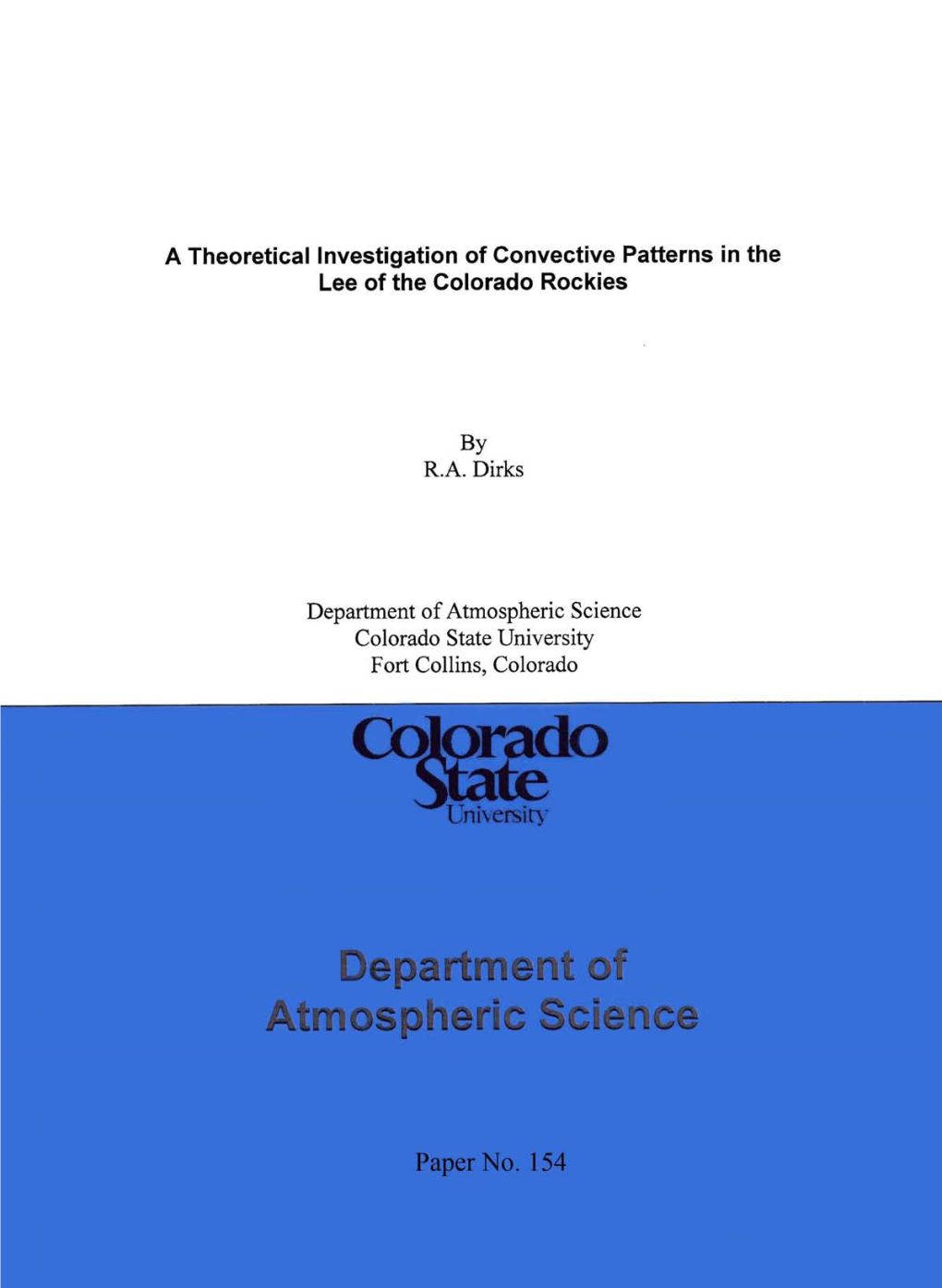
Load more
Recommended publications
-
Summits on the Air – ARM for USA - Colorado (WØC)
Summits on the Air – ARM for USA - Colorado (WØC) Summits on the Air USA - Colorado (WØC) Association Reference Manual Document Reference S46.1 Issue number 3.2 Date of issue 15-June-2021 Participation start date 01-May-2010 Authorised Date: 15-June-2021 obo SOTA Management Team Association Manager Matt Schnizer KØMOS Summits-on-the-Air an original concept by G3WGV and developed with G3CWI Notice “Summits on the Air” SOTA and the SOTA logo are trademarks of the Programme. This document is copyright of the Programme. All other trademarks and copyrights referenced herein are acknowledged. Page 1 of 11 Document S46.1 V3.2 Summits on the Air – ARM for USA - Colorado (WØC) Change Control Date Version Details 01-May-10 1.0 First formal issue of this document 01-Aug-11 2.0 Updated Version including all qualified CO Peaks, North Dakota, and South Dakota Peaks 01-Dec-11 2.1 Corrections to document for consistency between sections. 31-Mar-14 2.2 Convert WØ to WØC for Colorado only Association. Remove South Dakota and North Dakota Regions. Minor grammatical changes. Clarification of SOTA Rule 3.7.3 “Final Access”. Matt Schnizer K0MOS becomes the new W0C Association Manager. 04/30/16 2.3 Updated Disclaimer Updated 2.0 Program Derivation: Changed prominence from 500 ft to 150m (492 ft) Updated 3.0 General information: Added valid FCC license Corrected conversion factor (ft to m) and recalculated all summits 1-Apr-2017 3.0 Acquired new Summit List from ListsofJohn.com: 64 new summits (37 for P500 ft to P150 m change and 27 new) and 3 deletes due to prom corrections. -
Great Sand Dunes National Park and Preserve Foundation Document
NATIONAL PARK SERVICE • U.S. DEPARTMENT OF THE INTERIOR Foundation Document Great Sand Dunes National Park and Preserve Colorado January 2017 Foundation Document Crestone Colorado Road T To 17 C a m in o B a ca G r an de l a Crestone Peak e R 14294ft o 4357m n i m a C Marble Mountain 13266ft 4043m W To 69 a Creek g o n Milwaukee Peak Falls W 13522ft h 4122m Crystal e e 119 l R SAN ISABEL o a d Creek NATIONAL od nwo tto Co Upper FOREST ey Ca Sand Creek el R m D in Music Mountain Lake ino o 13355ft m 11745ft Ca B 4071m Creek a 3580m c Creek a S Pass G r a RIO GRANDE A n Music Pass d Lower 11380ft Music e NATIONAL N Sand Creek Lake 3469m PRIVATE PROPERTY 11473ft G 3497m Grape FOREST Tijeras Peak 13604ft d a R 4146m Creek o R y E t r Snowslide Mountain e 11664 ft b i 3555m L Deadman Lakes D Cottonwood Cleveland Peak E Liberty Gate 13414ft Blueberry Peak (backcountry access) 4089m 12005ft 3659m Little Sand Creek Lakes C R North Muddy k Cree I S Creek k e C e an reek Alpine r Deadm C T O le Po M Hudson Branch O ek re C U rt N To 69 Sho Sand ek re C T 559 A Medano Lake Medano Pass I 11518ft 9982ft STORM WINDS 3511m 3043m N Mount Herard 13297ft 4053m S WATER GREAT SAND DUNES Creek NATIONAL PRESERVE Bruff Sand Creek Ra Middle mp Tr ail Cold National Preserve BACA National Park Many primitive campsites along road in this area. -
Geology of Pre-Tertiary Rocks in the Northern Part of Yellowstone National Park, Wyon1ing by EDWARD T
GEOLOGY OF PRE- TERTIARY ROCKS IN THE NORTHERN PART OF YELLOWSTONE NATIONAL PARK, m WYOMING "* ,~ - DivMOri Coforado Distril'l r ih•." n' Geology of Pre-Tertiary Rocks in the Northern Part of Yellowstone National Park, Wyon1ing By EDWARD T. RUPPEL With a section on Tertiary laccoliths, sills, and stocks in and near the Gallatin Range, Yellowstone National Park GEOLOGY OF YELLOWSTONE NATIONAL PARK GEOLOGICAL SURVEY PROFESSIONAL PAPER 729-A UNITED STATES GOVERNMENT PRINTING OFFICE, WASHINGTON : 1972 UNITED STATES DEPARTMENT OF THE INTERIOR ROGERS C. B. MORTON, Secretary GEOLOGICAL SURVEY V. E. McKelvey, Director Library of Congress catalog-card No. 72-600268 For sale by the Superintendent of Documents, U.S. Government Printing Office Washington, D.C. 20402 Stock Number 2401-00242 Yellowstone National Park, the oldest of the areas set aside as part of the national park sys tem, lies amidst the Rocky Mountains in north western Wyoming and adjacent parts of Montana and Idaho. Embracing large, diverse, and complex geologic features, the park is in an area that is critical to the interpretation of many significant regional geologic problems. In order to provide basic data bearing on these problems, the U.S. Geological Survey in 1965 initiated a broad pro gram of comprehensive geologic and geophysical investigations within the park. This program was carried out with the cooperation of the National Park Service, and was also aided by the National Aeronautics and Space Administration, which supported the gathering of geologic information needed in testing and in interpreting results from various remote sensing devices. This professional paper chapter is one of a series of technical geo logic reports resulting from these investigations. -
Jim Mcclure-Jerry Peak Wilderness Management Plan
NATIONAL SYSTEM OF PUBLIC LANDS U.S. DEPARTMENT OF THE INTERIOR BUREAU OF LAND MANAGEMENT United States Department of Agriculture United States Department of the Interior Forest Service Bureau of Land Management Jim McClure-Jerry Peak Wilderness Management Plan Salmon-Challis National Forest BLM, Idaho Falls District, Challis Field Office February 10, 2017 For More Information Contact: Charles A. Mark, Forest Supervisor Salmon-Challis National Forest 1206 S. Challis Street Salmon, ID 83467 Phone: 208-756-5100 Fax: 208-737-3236 Mary D’Aversa, District Manager Idaho Falls District 1405 Hollipark Drive Idaho Falls, ID 83401 Phone: 208-524-7500 Fax: 208-737-3236 Description: Jim McClure-Jerry Peak Wilderness USDA NON-DISCRIMINATION POLICY STATEMENT DR 4300.003 USDA Equal Opportunity Public Notification Policy (June 2, 2015) In accordance with Federal civil rights law and U.S. Department of Agriculture (USDA) civil rights regulations and policies, the USDA, its Agencies, offices, and employees, and institutions participating in or administering USDA programs are prohibited from discriminating based on race, color, national origin, religion, sex, gender identity (including gender expression), sexual orientation, disability, age, marital status, family/parental status, income derived from a public assistance program, political beliefs, or reprisal or retaliation for prior civil rights activity, in any program or activity conducted or funded by USDA (not all bases apply to all programs). Remedies and complaint filing deadlines vary by program or incident. Persons with disabilities who require alternative means of communication for program information (e.g., Braille, large print, audiotape, American Sign Language, etc.) should contact the responsible Agency or USDA’s TARGET Center at (202) 720-2600 (voice and TTY) or contact USDA through the Federal Relay Service at (800) 877-8339. -
The Rambler April 2000 Volume 77 Number 4
The Rambler April 2000 Volume 77 Number 4 / / Page2 The Rambler April 2000 Managing Editor: Jeanette Buenger TO SUBMIT AN ARTICLE: Articles on trip talks or other Advertising Coordinator: Jaylene Myrup topics of general interest are welcome. Articles may be (583-1678) submitted by: Mailing Coordinator: Chris Venizelos 1 . Email submission as an attachment to (364-4544) [email protected]. 2. Mail submissions to the Publications Director at the The Rambler (USPS 053-410) is published by the office address. Wasatch Mountain Club, Inc., 1390 South 1100 East, 3. Hand delivered to the WMC office (M-F, 8-5.) Suite 103, Salt Lake City, UT, 84105-2461, (463-9842.) Deliveries can be left in the blue box outside the Subscription rates of $12.00 per year are paid for by office door. membership dues only. Periodicals Postage Paid at Salt Lake City, Utah. HOW TO SUBMIT TO THE RAMBLER: • Submit your articles on a 3.5" diskette in MS POSTMASTER: Send address changes to The Wordpad, MS Word, or WordPerfect format. Rambler, Membership Director, 1390 South 1100 East, • Use Arial font, 10 point for all submissions. For Salt Lake City, UT 84105-2443. CHANGE OF activity submissions, do not use any special ADDRESS: This publication is not forwarded by the Post formatting other than bold. Office. • Label the disk with your name and identify which files are submissions. The right is reserved to edit all contributions and • Attach a hard copy in case of problems. advertisements, and to reject those that may harm the • The deadline is 6:00 p.m. -
Challis National Forest
P a Tendoy S n Creek D h econd c ev a t S M i Taylor Mountain D tt rk Lemhi Pass h eer C ey e k C r k C Cr S r s Cr e e e e t e e r e Lo e e Cr o ek i e Degan Mountain e k tt Agency Creek w l k k i C r R ev v ek d cD ig Dry C e r r C Iron Creek I e M B G ee r k r e ro C u r n l k ng c e C e C i k e r h e k re p Poison Peak Tendoy e r y e S e C r k m r n e o ar Goldbug Ridge dy C ia e G M d Nort W o u ar k M h F o e ork Ha s Y an t e eari C C Y r reek Poison Creek C k e Opal Lake r C r in r e e Cab o k ek e e F e k il C e h r R ra r k t H in T eek u C gle C o k reek 30 S a 28 in 28 t eek k asin Cr Hayden Creek C B e M e Wards Butte r r Sh k L Goat Mountain e e C p k k e e C e ee e m astle Allison Creek r e e e k Creek eek k C C r r h s e k Big Hat Cr r r C e e e e ea C i e r e e Creek Lem Peak B y R R k r k McKim Lemhi C e lder Cree Hat Creek r n i re Bannock Pass A k C B D v n ea e e C l M o o P k r Val d r s e a o a le l Black Mountain se r Creek e y y r i k r k Hat e C a e m ee r C r r t t r o r C n ee k e h k e re o Creek C k H e P g e C T C e e is k i e k l e e e c k l k k d a r k A e r E r rn a c e r Fu il e eek e k e R r u r C C e C e k C l C L at t h r C e e c e r e H B r h n t l i e l H t p itt h e i u n c k L e Z o C k ig C s L G k a e r d r e or r s o C t r W ie C F e ll n y r n C w C tt k e o k o n e a r a e o k e Cow Creek C P e y n e e M a g e k n W k e r k r O r k e S C e e e l a s d C t d t o e R e e C a H 29 y l r r t C d e w r 29 F l org i C e H y o M E M a r C s r C l Leadore 100k a e l w 2 k n t i 8 e k k li r M l y -
Article Title: Perkey's Names of Nebraska Locations
Nebraska History posts materials online for your personal use. Please remember that the contents of Nebraska History are copyrighted by the Nebraska State Historical Society (except for materials credited to other institutions). The NSHS retains its copyrights even to materials it posts on the web. For permission to re-use materials or for photo ordering information, please see: http://www.nebraskahistory.org/magazine/permission.htm Nebraska State Historical Society members receive four issues of Nebraska History and four issues of Nebraska History News annually. For membership information, see: http://nebraskahistory.org/admin/members/index.htm Article Title: Perkey’s Names of Nebraska Locations Full Citation: Elton A Perkey, “Perkey’s Names of Nebraska Locations,” Nebraska History 59 (1978): 259-297. URL of article: http://www.nebraskahistory.org/publish/publicat/history/full-text/NH1978PerkeysHamilton-Merrick.pdf Date: 1/6/2015 Article Summary: This is a continuation of the serial publication of Perkey’s Names, organized by County, continued from the Spring Issue, 1978. This represents Hamilton County through Merrick County, Nebraska. PERKEY'S NAMES OF NEBRASKA LOCATIONS By Elton A. Perkey (Continued from Spring Issue. 1978. Following its serial publication in Nebraska History. it is planned to publish the entire listing of "Perkey's Names," with anv additions or corrections. in book form.) . HAMILTON COUNTY Named in honor of Alexander Hamilton (1757·1804). secretary of the treasury in President George Washington's Cabinet. Boundaries of the county were defined by an act approved February 16, 1867. A1Yln. Post office name changed from Hamilton January 10, 1876; discontinued February 23, 1888. Origin of the name not learned. -
The Buffalo River: a Jurisprudence of Preservation, 21 B.C
Boston College Environmental Affairs Law Review Volume 21 | Issue 3 Article 2 5-1-1994 The uB ffalo River: A Jurisprudence of Preservation John W. Ragsdale, Jr. Follow this and additional works at: http://lawdigitalcommons.bc.edu/ealr Part of the Environmental Law Commons, and the Jurisprudence Commons Recommended Citation John W. Ragsdale, Jr., The Buffalo River: A Jurisprudence of Preservation, 21 B.C. Envtl. Aff. L. Rev. 429 (1994), http://lawdigitalcommons.bc.edu/ealr/vol21/iss3/2 This Article is brought to you for free and open access by the Law Journals at Digital Commons @ Boston College Law School. It has been accepted for inclusion in Boston College Environmental Affairs Law Review by an authorized editor of Digital Commons @ Boston College Law School. For more information, please contact [email protected]. THE BUFFALO RIVER: A JURISPRUDENCE OF PRESERVATION John W Ragsdale, Jr. * In the middle of the country lie the Ozark Mountains, islands of green and rumpled respite from the human-dominated sameness of the surrounding plains. These lower-case mountains, though less im posing than their eastern or western counterparts, are nonetheless interesting, exciting and often untamed. There is particular wildness and isolation in the hollows because the highways, unlike those in the western mountains, have tended to run on the ridge crests. The crystal streams in these roadless valleys, though usually calmer than the snow-fed chargers of the Colorado Rockies, still have numerous rapids and shoals whose allure is enhanced by their solitude. At the center of this verdant repose of highlands, forest and waters is the Buffalo River, a free-flowing stream throughout its length and a place of incomparable beauty. -
University Microfilms, Inc., Ann Arbor, Michigan the DEVONIAN SYSTEM in WESTERN WYOMING
This dissertation has been 65—13,200 microfilmed exactly as received BENSON, Anthony Lane, 1939- THE DEVONIAN SYSTEM IN WESTERN WYOMING AND ADJACENT AREAS. The Ohio State University, Ph.D., 1965 Geology University Microfilms, Inc., Ann Arbor, Michigan THE DEVONIAN SYSTEM IN WESTERN WYOMING AND ADJACENT AREAS DISSERTATION Presented in Partial Fulfillment of the Requirements for the Degree Doctor of Philosophy in the Graduate School of Hie Ohio State University By Anthony Lane Benson, B. S The Ohio State University 1965 Approved by A dviser Department of Geology This dissertation has boon 05—13,200 microfilmed exactly as received BENSON, Anthony Lane, 1939— THE DEVONIAN SYSTEM IN WESTERN WYOMING AND ADJACENT AREAS. The Ohio State University, Ph.D., 1905 Geology University Microfilms, Inc., Ann Arbor, Michigan THE DEVONIAN SYSTEM IN WESTERN WYOMING AND ADJACENT AREAS DISSERTATION Presented In Partial Fulfillment of the Regulresents for the Degree Doctor of Riilosophy In the Graduate School of Uie Ohio State University By Anthony lane Benson, B. S lhe Ohio State University 1965 Approved by A dviser Department of Geology VITA Noveetoer 1939 Born - Cleveland, Ohio 1961 B. S., The Ohio State tfelverslty, Coluribus, Ohio 1961 Field Assistant, United States Geological Survey, Denver, Colorado 196I- 196U Graduate Teaching Assistant, The Ohio Stats Uhlverslty, Coluntme, Ohio 196^ Geologist, The California Company, Jackson, Klssisslppl 1965 Instructor, The Ohio State Uhlverslty, Lina Branch, U na, Ohio 1965- Geologist, Itan American Petroleum Corporation, Oklahoma City, Qklahosm 11 CONTENTS introduction............................................................................ 1 Purpose and Scope 1 P revious Work 5 Methods of Investigation 7 Acknowledgments 7 Nomenclature 8 MAYWOOD FORMATION.............................................................. -
Development of a North American Survey for Monitoring Shorebird Populations
DEVELOPMENT OF A NORTH AMERICAN SURVEY FOR MONITORING SHOREBIRD POPULATIONS Report to: U.S. Fish and Wildlife Service Patuxent Wildlife Research Center Laurel, MD 20708 Prepared by: Brian A. Barrington Gary W. Page Manomet Bird Observatory Point Reyes Bird Observatory P.O. Box 1770 4990 Shoreline Highway Manomet MA 02345 Stinson Beach CA 94970 Assisted by; James E. Lyons & Janet E. Kjelmyr & u. Cartar w. David Shuford Manomet Bird Observatory Point Reyes Bird Observatory September 1991 1 SYNOPSIS Tasks The purpose of this report is to investigate the feasibility of a survey for North American shorebird populations that will provide reliable annual indices to population sizes of as many species as possible and allow analysis of long-term population changes to be conducted. Specific tasks were: 1. To identify all known spring and fall migration stopovers in North America for which a typical migration peak is at least 5000 shorebirds and to provide information on ownership, accessibility, special logistical considerations and habitat stability. (We added to this the identification of all sites likely to hold 5000 or more shorebirds during winter.) 2. To propose a sampling protocol that focuses on the identified sites and considers census frequency, best time of year for counts, ef feet of migration timing on counts, · and need for team census approach. The potential effectiveness of the sampling protocol must be analyzed for all species of North American shorebirds. The sampling protocol must include a rational for omitting any significant -
Yellowstone Center for Resources
YELLOWSTONE CENTER FOR RESOURCES IAL Ana SPAT lysis s e N c SOURC r RE E A u T o U s e R R A L L R A e R s o U u T r L c e U s C I nformation R ES rt EARCH Suppo ANNUAL2002 REPORT FISCAL YEAR YELLOWSTONE CENTER FOR RESOURCES 2002 ANNUAL REPORT FISCAL YEAR (OCTOBER 1, 2001, TO SEPTEMBER 30, 2002) Yellowstone Center for Resources National Park Service Yellowstone National Park, Wyoming YCR–AR–2002 2003 Suggested citation: Yellowstone Center for Resources. 2003. Yellowstone Center for Resources Annual Report, Fiscal Year 2002. National Park Service, Mammoth Hot Springs, Wyoming, YCR–AR–2002. Photographs not otherwise marked are courtesy of the National Park Service. Front cover: clockwise from top right, a possible McKean component precontact site; J.E. Stuart’s “Old Faithful” oil painting; elk and a Druid pack wolf by Monty Sloan; Eastern Shoshone dancer by Sandra Nykerk; and center, peregrine falcon fledgling by Wayne Wolfersberger. Title page: wolf #113, alpha male of the Agate Creek pack, attacking a cow elk. Photo by anonymous donor. Back cover: Yellowstone cutthroat trout. ii Contents Introduction..........................................................................................................iv Part I. Resource Highlights ...............................................................................1 Part II. Cultural Resource Programs ................................................................7 Archeology.......................................................................................................8 Ethnography -
PDF Maps App N O
114°40'0"W 114°20'0"W 114°0'0"W FRANK CHURCH - RIVESRh eOrmF aNnO P eRakETURN WILDERNESS Mayfield Peak Corkscrew Mountain " " " d L CASTO o SHERMAN PEAK CHALLIS CREEK LAKES TWIN PEAKS PATS CREEK R on CHALLIS h GROUSE PEAK MAY EAST OF MAY C Mosquito Flat Reservoir lc re ek u ek re G R ll C Challis n d i !( to M a e d L d d s R R g 3 R d 1 n R i 1 k r d e k p a e e o r S p R e velo M C e e mile ll r Blue Mountain rest D t i o g igh C C Natl F d E r N n U i e e " S Meadow Peak e R k f rd H Table Mountain Estes Mountain T G a w R r y " " d a 9 " il 3 1 MOUNT JORDAN 7 CUSTER ELEVENMILE CREEK 2 BAYHORSE LAKE KBeyAsYtoHneO MRoSunEtain BRADBURY FLAT Unnamed Lake MEADOW PEAK " Ramshorn Mountain LITTLE ANTELOPE FLAT d " R k or F Rd Bald Mountain e B k ke ay e ho d re " an rse C C Y reek R Lim e Rd ld O Lucky B oy Rd R t Bachelor Mountain d a S Bald Mountain l " F Grouse Creek Mountain q l Bonanza Peak u " ia " a n Nf 0 4 ente " w 5 C C R Lone Pine Peak d N r e " e " 0 k ' R 0 2 d ° Nf 4 37 4 2 R d EAST BASIN CREEK SUNBEAM THOMPSON CREEK CLAYTON BALD MOUNTAIN LONE PINE PEAK ANTELOPE FLAT GROUSE CREEK MOUNTAIN T h o m ps on C r Saturday Mountain R d e on e y k " n a R C ree d r p C k R a Shee d 75 Sp 93 Q Sta wy 75 6 ¤£ R t H d 6 e a 6 R o d R p lo e v Dickey Peak e D Potaman Peak " t s Sullivan Lake " e Robinson Bar Peak r o F " l t Anderson Peak Lookout Mountain a N " " CASINO LAKES ROBINSON BAR LIVINGSTON CREEK POTAMAN PEAK ZIEGLER BASIN THE PAINT POT HORSE BASIN DICKEY PEAK S A L M O N - C H A L L I S N F U S R Jimmy Smith Lake o H ad w Cr y