GUN1 Regulates Tetrapyrrole Biosynthesis
Total Page:16
File Type:pdf, Size:1020Kb
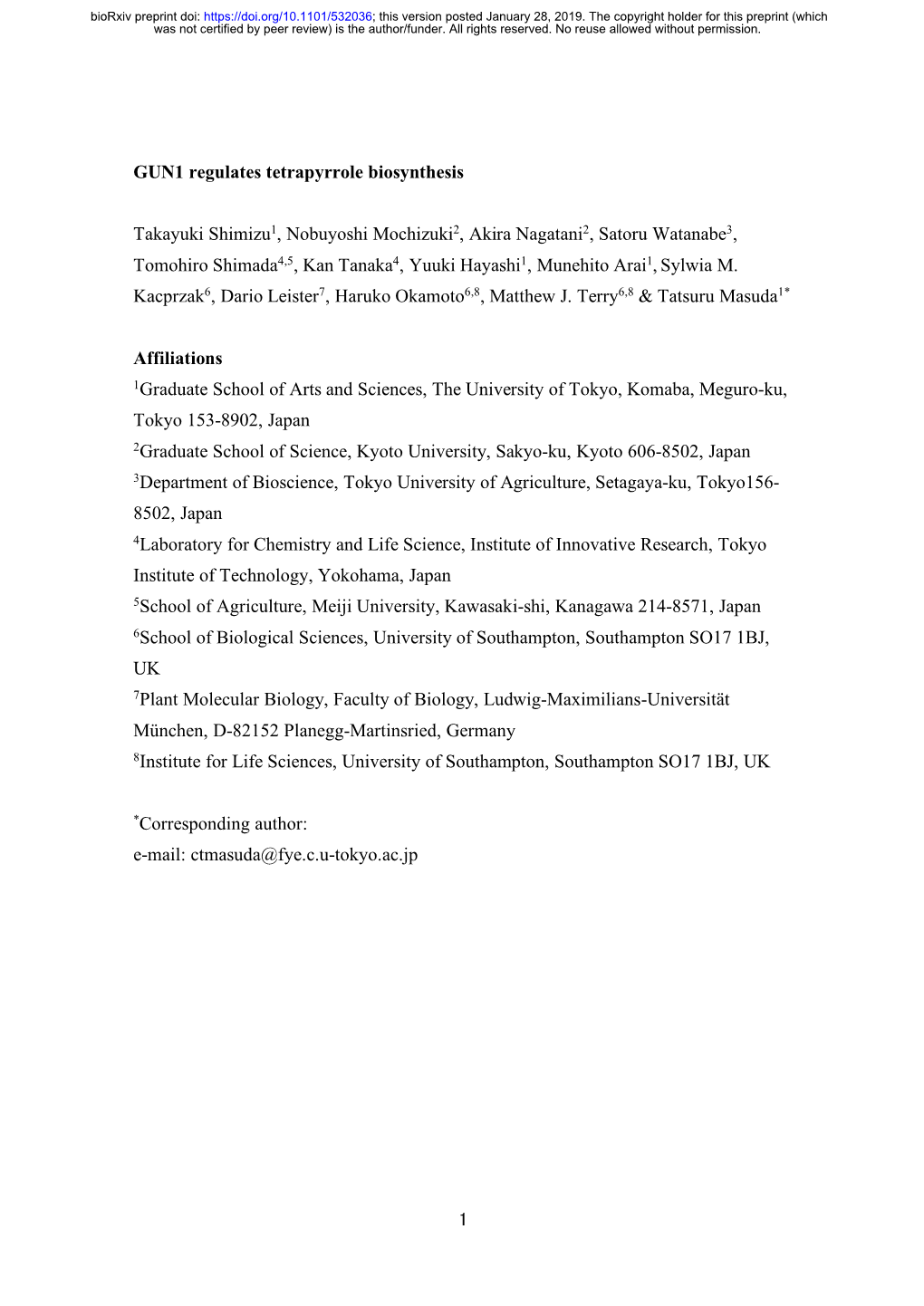
Load more
Recommended publications
-
Magnesium-Protoporphyrin Chelatase of Rhodobacter
Proc. Natl. Acad. Sci. USA Vol. 92, pp. 1941-1944, March 1995 Biochemistry Magnesium-protoporphyrin chelatase of Rhodobacter sphaeroides: Reconstitution of activity by combining the products of the bchH, -I, and -D genes expressed in Escherichia coli (protoporphyrin IX/tetrapyrrole/chlorophyll/bacteriochlorophyll/photosynthesis) LUCIEN C. D. GIBSON*, ROBERT D. WILLOWSt, C. GAMINI KANNANGARAt, DITER VON WETTSTEINt, AND C. NEIL HUNTER* *Krebs Institute for Biomolecular Research and Robert Hill Institute for Photosynthesis, Department of Molecular Biology and Biotechnology, University of Sheffield, Sheffield, S10 2TN, United Kingdom; and tCarlsberg Laboratory, Department of Physiology, Gamle Carlsberg Vej 10, DK-2500 Copenhagen Valby, Denmark Contributed by Diter von Wettstein, November 14, 1994 ABSTRACT Magnesium-protoporphyrin chelatase lies at Escherichia coli and demonstrate that the extracts of the E. coli the branch point of the heme and (bacterio)chlorophyll bio- transformants can convert Mg-protoporphyrin IX to Mg- synthetic pathways. In this work, the photosynthetic bacte- protoporphyrin monomethyl ester (20, 21). Apart from posi- rium Rhodobacter sphaeroides has been used as a model system tively identifying bchM as the gene encoding the Mg- for the study of this reaction. The bchH and the bchI and -D protoporphyrin methyltransferase, this work opens up the genes from R. sphaeroides were expressed in Escherichia coli. possibility of extending this approach to other parts of the When cell-free extracts from strains expressing BchH, BchI, pathway. In this paper, we report the expression of the genes and BchD were combined, the mixture was able to catalyze the bchH, -I, and -D from R. sphaeroides in E. coli: extracts from insertion of Mg into protoporphyrin IX in an ATP-dependent these transformants, when combined in vitro, are highly active manner. -
AOP 131: Aryl Hydrocarbon Receptor Activation Leading to Uroporphyria
Organisation for Economic Co-operation and Development DOCUMENT CODE For Official Use English - Or. English 1 January 1990 AOP 131: Aryl hydrocarbon receptor activation leading to uroporphyria Short Title: AHR activation-uroporphyria This document was approved by the Extended Advisory Group on Molecular Screening and Toxicogenomics in June 2018. The Working Group of the National Coordinators of the Test Guidelines Programme and the Working Party on Hazard Assessment are invited to review and endorse the AOP by 29 March 2019. Magdalini Sachana, Administrator, Hazard Assessment, [email protected], +(33- 1) 85 55 64 23 Nathalie Delrue, Administrator, Test Guidelines, [email protected], +(33-1) 45 24 98 44 This document, as well as any data and map included herein, are without prejudice to the status of or sovereignty over any territory, to the delimitation of international frontiers and boundaries and to the name of any territory, city or area. 2 │ Foreword This Adverse Outcome Pathway (AOP) on Aryl hydrocarbon receptor activation leading to uroporphyria, has been developed under the auspices of the OECD AOP Development Programme, overseen by the Extended Advisory Group on Molecular Screening and Toxicogenomics (EAGMST), which is an advisory group under the Working Group of the National Coordinators for the Test Guidelines Programme (WNT). The AOP has been reviewed internally by the EAGMST, externally by experts nominated by the WNT, and has been endorsed by the WNT and the Working Party on Hazard Assessment (WPHA) in xxxxx. Through endorsement of this AOP, the WNT and the WPHA express confidence in the scientific review process that the AOP has undergone and accept the recommendation of the EAGMST that the AOP be disseminated publicly. -
On Tuning the Fluorescence Emission of Porphyrin Free Bases Bonded to the Pore Walls of Organo-Modified Silica
Molecules 2014, 19, 2261-2285; doi:10.3390/molecules19022261 OPEN ACCESS molecules ISSN 1420-3049 www.mdpi.com/journal/molecules Article On Tuning the Fluorescence Emission of Porphyrin Free Bases Bonded to the Pore Walls of Organo-Modified Silica Rosa I. Y. Quiroz-Segoviano 1, Iris N. Serratos 1, Fernando Rojas-González 1, Salvador R. Tello-Solís 1, Rebeca Sosa-Fonseca 2, Obdulia Medina-Juárez 1, Carmina Menchaca-Campos 3 and Miguel A. García-Sánchez 1,* 1 Departamento de Química, Universidad Autónoma Metropolitana-Iztapalapa, Av. San Rafael Atlixco 186, Vicentina, D. F. 09340, Mexico 2 Departamento de Física, Universidad Autónoma Metropolitana-Iztapalapa, Av. San Rafael Atlixco 186, Vicentina, D. F. 09340, Mexico 3 Centro de Investigación en Ingeniería y Ciencias Aplicadas, UAEM, Av. Universidad 1001, Col. Chamilpa, C.P. 62209, Cuernavaca Mor., Mexico * Author to whom correspondence should be addressed; E-Mail: [email protected]; Tel.: +52-55-5804-4677; Fax: +52-55-5804-4666. Received: 24 December 2013; in revised form: 29 January 2014 / Accepted: 7 February 2014 / Published: 21 February 2014 Abstract: A sol-gel methodology has been duly developed in order to perform a controlled covalent coupling of tetrapyrrole macrocycles (e.g., porphyrins, phthalocyanines, naphthalocyanines, chlorophyll, etc.) to the pores of metal oxide networks. The resulting absorption and emission spectra intensities in the UV-VIS-NIR range have been found to depend on the polarity existing inside the pores of the network; in turn, this polarization can be tuned through the attachment of organic substituents to the tetrapyrrrole macrocycles before bonding them to the pore network. -
Preparation of Tetrapyrrole-Amino Acid Covalent Complexes
I'lunt I'ht.siol.Ritx ltt'nt. 1996. -14 (3). 393-39lt Preparation of tetrapyrrole-amino acid covalent complexes Leszek Fiedorl'2*, Varda Rosenbach-Belkinl, Maruthi Sail and Avigdor Scherzl I BiochernistryDepartment. The Weizn-rannInstitute of Science.76100 Rehovot.Israel. I Prcscntaddress: Institute of Molecular BiologSr.Ja-ciellonian University. Al. Mickiewicza 3. 3 l- 120 Cracow. Poland. ':'Author to whom correspondenceshould bc addrcsscd(fax +48-12-336907:E-mail fiedor@)mol.uj.edu.pl) Abstract The presentedsynthetic approach towards chcn'rical modifications of chlorophylls(Chls) provides a perspectivcto construct model systems. where tetrapyrrole-aminoacid and tetrapyrrole-peptideinteractions coulcl be studied in covalent rnodel compor,rncls. The approach relies on thc lact that in Chls the | 7r propionic rcid sidc chain docs not participatc in the tetrapl'rroleii--electron system. It makes use of a plant enzvmechlolophyllase (EC 3.1.1.1,+).which lrr lilo and in yitrc catalysesreactions at this sidc function. The transesterilicationand hyclrolysisenzymatic rerctions are useful on a preparativescale. ln the transesterificationreaction. a desiredamino acid rcsiduc posscssirrgprimary hydloxyl group can be directly attachedto the propiorric acid side chain o1' Chl. This mcthod allows to replace the phytyl moiety in Chls n'ith seline. The r:rtherreaction. enzyrratic hydrolysis of Chls, yields chlorophyllides and opens a convenientroutc fbr furthcr rnodifications.If sufliciently mild synthetic mcthodsarc uscd. such as catalysisw,ith ,l-dimethyl arnino pyridine or activationwith N-hvdroxvsuccinimide.an arrino acid or peptide residuecan be covalentlybound to chlorophyllides' carboxylic group. lear,'ingthe essentialclectlonic structure of Chl intact. The activation w'ith N-hydroxvsuccininridcallows fbr the coupling cvrn in aqueous rncdia. -
Continuous Chlorophyll Degradation Accompanied by Chlorophyllide and Phytol Reutilization for Chlorophyll Synthesis in Synechocystis Sp
View metadata, citation and similar papers at core.ac.uk brought to you by CORE provided by Elsevier - Publisher Connector Biochimica et Biophysica Acta 1767 (2007) 920–929 www.elsevier.com/locate/bbabio Continuous chlorophyll degradation accompanied by chlorophyllide and phytol reutilization for chlorophyll synthesis in Synechocystis sp. PCC 6803 ⁎ Dmitrii Vavilin, Wim Vermaas School of Life Sciences and Center for the Study of Early Events in Photosynthesis, Arizona State University, Box 874501, Tempe, AZ 85287, USA Received 3 January 2007; received in revised form 23 March 2007; accepted 27 March 2007 Available online 3 April 2007 Abstract Chlorophyll synthesis and degradation were analyzed in the cyanobacterium Synechocystis sp. PCC 6803 by incubating cells in the presence of 13C-labeled glucose or 15N-containing salts. Upon mass spectral analysis of chlorophyll isolated from cells grown in the presence of 13C-glucose for different time periods, four chlorophyll pools were detected that differed markedly in the amount of 13C incorporated into the porphyrin (Por) and phytol (Phy) moieties of the molecule. These four pools represent (i) unlabeled chlorophyll (12Por12Phy), (ii) 13C-labeled chlorophyll (13Por13Phy), and (iii, iv) chlorophyll, in which either the porphyrin or the phytol moiety was 13C-labeled, whereas the other constituent of the molecule remained unlabeled (13Por12Phy and 12Por13Phy). The kinetics of 12Por12Phy disappearance, presumably due to chlorophyll de- esterification, and of 13Por12Phy, 12Por13Phy, and 13Por13Phy accumulation due to chlorophyll synthesis provided evidence for continuous chlorophyll turnover in Synechocystis cells. The loss of 12Por12Phy was three-fold faster in a photosystem I-less strain than in a photosystem II- less strain and was accelerated in wild-type cells upon exposure to strong light. -
Bilirubin Suppresses Th17 Immunity in Colitis by Upregulating CD39
Bilirubin suppresses Th17 immunity in colitis by upregulating CD39 Maria Serena Longhi, … , Francisco J. Quintana, Simon C. Robson JCI Insight. 2017;2(9):e92791. https://doi.org/10.1172/jci.insight.92791. Research Article Gastroenterology Immunology Unconjugated bilirubin (UCB), a product of heme oxidation, has known immunosuppressant properties but the molecular mechanisms, other than antioxidant effects, remain largely unexplored. We note that UCB modulates T helper type 17 (Th17) immune responses, in a manner dependent upon heightened expression of CD39 ectonucleotidase. UCB has protective effects in experimental colitis, where it enhances recovery after injury and preferentially boosts IL-10 production by colonic intraepithelial CD4+ cells. In vitro, UCB confers immunoregulatory properties on human control Th17 cells, as reflected by increased levels of FOXP3 and CD39 with heightened cellular suppressor ability. Upregulation of CD39 by Th17 cells is dependent upon ligation of the aryl hydrocarbon receptor (AHR) by UCB. Genetic deletion of CD39, as in Entpd1–/– mice, or dysfunction of AHR, as inA hrd mice, abrogates these UCB salutary effects in experimental colitis. However, in inflammatory bowel disease (IBD) samples, UCB fails to confer substantive immunosuppressive properties upon Th17 cells, because of decreased AHR levels under the conditions tested in vitro. Immunosuppressive effects of UCB are mediated by AHR resulting in CD39 upregulation by Th17. Boosting downstream effects of AHR via UCB or enhancing CD39-mediated ectoenzymatic activity might provide therapeutic options to address development of Th17 dysfunction in IBD. Find the latest version: https://jci.me/92791/pdf RESEARCH ARTICLE Bilirubin suppresses Th17 immunity in colitis by upregulating CD39 Maria Serena Longhi,1 Marta Vuerich,1 Alireza Kalbasi,1 Jessica E. -
Vitamin B, and B,-Proteins Edited by Bernhard Krautler, Duilio Arigoni and Bernard T
Vitamin B, and B,-Proteins Edited by Bernhard Krautler, Duilio Arigoni and Bernard T. Golding Lectures presented at the 4th European Symposium on Vitamin B,, and B,,-Proteins @ W I LEY-VCH Weinheim - Chichester - New York - Toronto. Brisbane - Singapore This Page Intentionally Left Blank Vitamin B, and BIZ-Proteins Edited by B. Krautler, D. Arigoni and B.T. Golding 633 WILEY-VCH This Page Intentionally Left Blank Vitamin B, and B,-Proteins Edited by Bernhard Krautler, Duilio Arigoni and Bernard T. Golding Lectures presented at the 4th European Symposium on Vitamin B,, and B,,-Proteins @ W I LEY-VCH Weinheim - Chichester - New York - Toronto. Brisbane - Singapore Prof. Dr. B. Krautler Prof. Dr. D. Arigoni Prof. Dr. B.T. Golding Leopold-Franzens-Universitat ETH-Zurich Department of Chemistry Innsbruck Laboratoriuin fur University of Newcastle Institut fur Organische Chemie Organische Chemie NE 17 RU Newcastle Iiinrain 52a Universitatsstrasse 16 upon Thyne A-6020 Innsbruck CH-8092 Zurich This book was carefully produced. Nevertheless, authors, editor and publisher do not warrant the in- formation contained theirein to be free of errors. Readers are advised to keep in mind that statements, data, illustrations, procedural details or other items may inadvertently be inaccurate. L I The cover picture shows a cartoon of B,,-dependent methionine synthase (see contribution by Drennan et al. in this book). The picture was kindly provided by Martin Tollinger, University of Innsbruck. Library of Congress Card No.: applied for British Library Cataloguing-in-Publication Data: A catalogue record for this book is available from the British Library Die Deutsche Bibliothek - CIP-Einheitsaufnahme Vitamin B,, and B,,-proteins :lectures presented at the 4th European Symposium on Vitamin B,, and B,,-Proteins / ed. -
Nomenclature of Tetrapyrroles
Pure & Appi. Chem. Vol.51, pp.2251—2304. 0033-4545/79/1101—2251 $02.00/0 Pergamon Press Ltd. 1979. Printed in Great Britain. PROVISIONAL INTERNATIONAL UNION OF PURE AND APPLIED CHEMISTRY and INTERNATIONAL UNION OF BIOCHEMISTRY JOINT COMMISSION ON BIOCHEMICAL NOMENCLATURE*t NOMENCLATURE OF TETRAPYRROLES (Recommendations, 1978) Prepared for publication by J. E. MERRITT and K. L. LOENING Comments on these proposals should be sent within 8 months of publication to the Secretary of the Commission: Dr. H. B. F. DIXON, Department of Biochemistry, University of Cambridge, Tennis Court Road, Cambridge CB2 1QW, UK. Comments from the viewpoint of languages other than English are encouraged. These may have special significance regarding the eventual publication in various countries of translations of the nomenclature finally approved by IUPAC-IUB. PROVISIONAL IUPAC—ITJB Joint Commission on Biochemical Nomenclature (JCBN), NOMENCLATUREOF TETRAPYRROLES (Recommendations 1978) CONTENTS Preface 2253 Introduction 2254 TP—O General considerations 2256 TP—l Fundamental Porphyrin Systems 1.1 Porphyrin ring system 1.2 Numbering 2257 1.3 Additional fused rings 1.4 Skeletal replacement 2258 1.5 Skeletal replacement of nitrogen atoms 2259 1.6Fused porphyrin replacement analogs 2260 1.7Systematic names for substituted porphyrins 2261 TP—2 Trivial names and locants for certain substituted porphyrins 2263 2.1 Trivial names and locants 2.2 Roman numeral type notation 2265 TP—3 Semisystematic porphyrin names 2266 3.1 Semisystematic names in substituted porphyrins 3.2 Subtractive nomenclature 2269 3.3 Combinations of substitutive and subtractive operations 3.4 Additional ring formation 2270 3.5 Skeletal replacement of substituted porphyrins 2271 TP—4 Reduced porphyrins including chlorins 4.1 Unsubstituted reduced porphyrins 4.2 Substituted reduced porphyrins. -
Indoleamine 2, 3-Dioxygenase Regulation of Immune Response (Review)
MOLECULAR MEDICINE REPORTS 17: 4867-4873, 2018 Indoleamine 2, 3-dioxygenase regulation of immune response (Review) HAO WU1, JIANPING GONG1 and YONG LIU2 1Chongqing Key Laboratory of Hepatobiliary Surgery and Department of Hepatobiliary Surgery, Second Affiliated Hospital, Chongqing Medical University, Chongqing 400010; 2Department of Hepatobiliary Surgery, The People's Hospital of Hechuan, Chongqing 401520, P.R. China Received November 28, 2016; Accepted January 4, 2018 DOI: 10.3892/mmr.2018.8537 Abstract. Indoleamine 2, 3-dioxygenase (IDO) catalyzes Contents the initial and rate-limiting step in the degradation pathway of the essential amino acid tryptophan and is expressed by 1. Introduction professional antigen presenting cells (APCs), epithelial cells, 2. Properties of the IDO enzyme vascular endothelium and tumor cells. IDO-mediated cata- 3. IDO pathways and immune regulation bolic products, which are additionally termed ʻkynureninesʼ, 4. Role of IDO in immune suppression exerts important immunosuppressive functions primarily via 5. Strategies to target IDO regulating T effector cell anergy and inducing the proliferation 6. Conclusions of T regulatory cells. This endogenous tolerogenic pathway has a critical effect on mediating the magnitude of immune responses under various stress conditions, including tumor, 1. Introduction infection and transplantation. The present review evaluates the recent progress in elucidating how catabolism of tryptophan As a highly evolved biological response, immunoregulation not regulated by IDO modulates the immune response to inflam- only coordinates inflammation and innate immunity, however matory and immunological signals. Blocking this pathway may may additionally modulate adaptive immunity and establish be a novel adjuvant therapeutic strategy for clinical application self-tolerance. Continuous access to nutrients is a primary in immunotherapy. -
The Marvels of Biosynthesis: Tracking Nature's Pathways
Pergamon Bioorganic & Medicinal Chemistry, Vol. 4, No. 7, pp 937-964, 1996 Copyright © 1996 Elsevier Science Ltd Printed in Great Britain. All rights reserved PIh S0968-0896(96)00102-2 0968-0896/96 $15.00+0.00 The Marvels of Biosynthesis: Tracking Nature's Pathways Alan R. Battersby University of Cambridge, University Chemical Laboratory, Lensfield Road, Cambridge CB2 1EW, U.K. Introduction and nitric acids, zinc, sulphur, copper sulphate and many more materials. Those days are gone and there How ever did it come about that a substantial part of are pluses and minuses to the change. At any rate, I my research has been aimed at understanding the was able to assemble a good set of equipment to run marvellous chemistry used by living systems to lots of simple experiments which I enjoyed enormously. ,:onstruct the substances they produce? I must admit ".hat I had not in the past thought much about that I believe the next important influence on me came at 9articular 'pathway' but was encouraged to do so by school where I had the great good fortune to be taught Derek Barton, Chairman of the Executive Board of more about chemistry by a superb teacher, Mr Evans. Editors for Tetrahedron Publications. He suggested The seed of my love for chemistry which had been that this article, invited by Professor Chi-Huey Wong, planted earlier by my father's books was strongly fed by should be a personal one giving some background on his teaching. Then I read my first books about organic how my interests evolved. -
Illuminating the Black Box of B12 Biosynthesis Harry A
COMMENTARY COMMENTARY Illuminating the black box of B12 biosynthesis Harry A. Dailey1 synthesis, one aerobic and the other anaer- Department of Microbiology, and Department of Biochemistry and Molecular Biology, obic. Whereas the genes required for both Biomedical and Health Sciences Institute, P.D. Coverdell Center, University of Georgia, Athens, aerobic and anaerobic synthesis have been GA 30602 known, the actual mechanism for synthesis via the anaerobic pathway has remained a large “black box” in what is one of the long- Tetrapyrroles are nearly ubiquitous in nature increasing or decreasing reactivity of the met- est known biosynthetic pathways. However, as participants in a wide variety of biological al center, and providing protection against the contents of this box have now been iden- reactions that are central to life, such as fi undesirable side reactions. One nds magne- tified and characterized, thanks to an elegant electron transfer, gas binding, and one-car- sium in chlorophyll, iron in hemes, nickel in study that can properly be called a tour de bon metabolism (1, 2). Because of their di- factor F430, and cobalt in cyano-cobalamin force by Moore et al. at the University of — verse colors for example the greens of plant (vitamin B12) (Fig. 1). Although hemes and Kent (3). chlorophylls, reds of blood, and blues and chlorophyls are synthesized by both prokar- Research into the metabolism of metallo- — browns of avian eggs tetrapyrroles have yotes and eukaryotes, factor F430,whichis tetrapyrroles and the biological and medical been called the “pigments of life.” The chem- involved in methanogenesis, is produced only impact of disordered synthesis or degradation ical diversity of cyclic tetrapyrroles owes by some archae, and cobalamin synthesis is of these compounds has been ongoing for the much to their ability to coordinate a variety found only in bacteria and archae. -
Destruction of Biological Tetrapyrrole Macrocycles by Hypochlorous Acid and Its Scavenging by Lycopene Dhiman Maitra Wayne State University
Wayne State University Wayne State University Dissertations 1-1-2011 Destruction of biological tetrapyrrole macrocycles by hypochlorous acid and its scavenging by lycopene Dhiman Maitra Wayne State University, Follow this and additional works at: http://digitalcommons.wayne.edu/oa_dissertations Part of the Biochemistry Commons, Nutrition Commons, and the Physiology Commons Recommended Citation Maitra, Dhiman, "Destruction of biological tetrapyrrole macrocycles by hypochlorous acid and its scavenging by lycopene" (2011). Wayne State University Dissertations. Paper 357. This Open Access Dissertation is brought to you for free and open access by DigitalCommons@WayneState. It has been accepted for inclusion in Wayne State University Dissertations by an authorized administrator of DigitalCommons@WayneState. DESTRUCTION OF BIOLOGICAL TETRAPYRROLE MACROCYCLE BY HYPOCHLOROUS ACID AND ITS SCAVENGING BY LYCOPENE by DHIMAN MAITRA DISSERTATION Submitted to the Graduate School of Wayne State University, Detroit, Michigan in partial fulfillment of the requirements for the degree of DOCTOR OF PHILOSPHY 2011 MAJOR: PHYSIOLOGY Approved by: _________________________________________ Advisor Date _____________________________________________ _____________________________________________ _____________________________________________ _____________________________________________ COPYRIGHT BY DHIMAN MAITRA 2011 All Rights Reserved DEDICATION To my parents and Shinjini for their love and support. ii ACKNOWLEDGEMENTS I would like to thank my advisor Dr. Husam M. Abu-Soud. Without his guidance and encouragement it would not have been possible for me to come this far. I would like to thank the members of my dissertation committee for their unending support. I would like to thank Drs. Michael P. Diamond and Ghasan M. Saed, Department of Obstetrics and Gynecology, Wayne State University, School of Medicine for their helpful comments and suggestions all through this work.