University of Groningen Diversity and Biocatalytic Potential Of
Total Page:16
File Type:pdf, Size:1020Kb
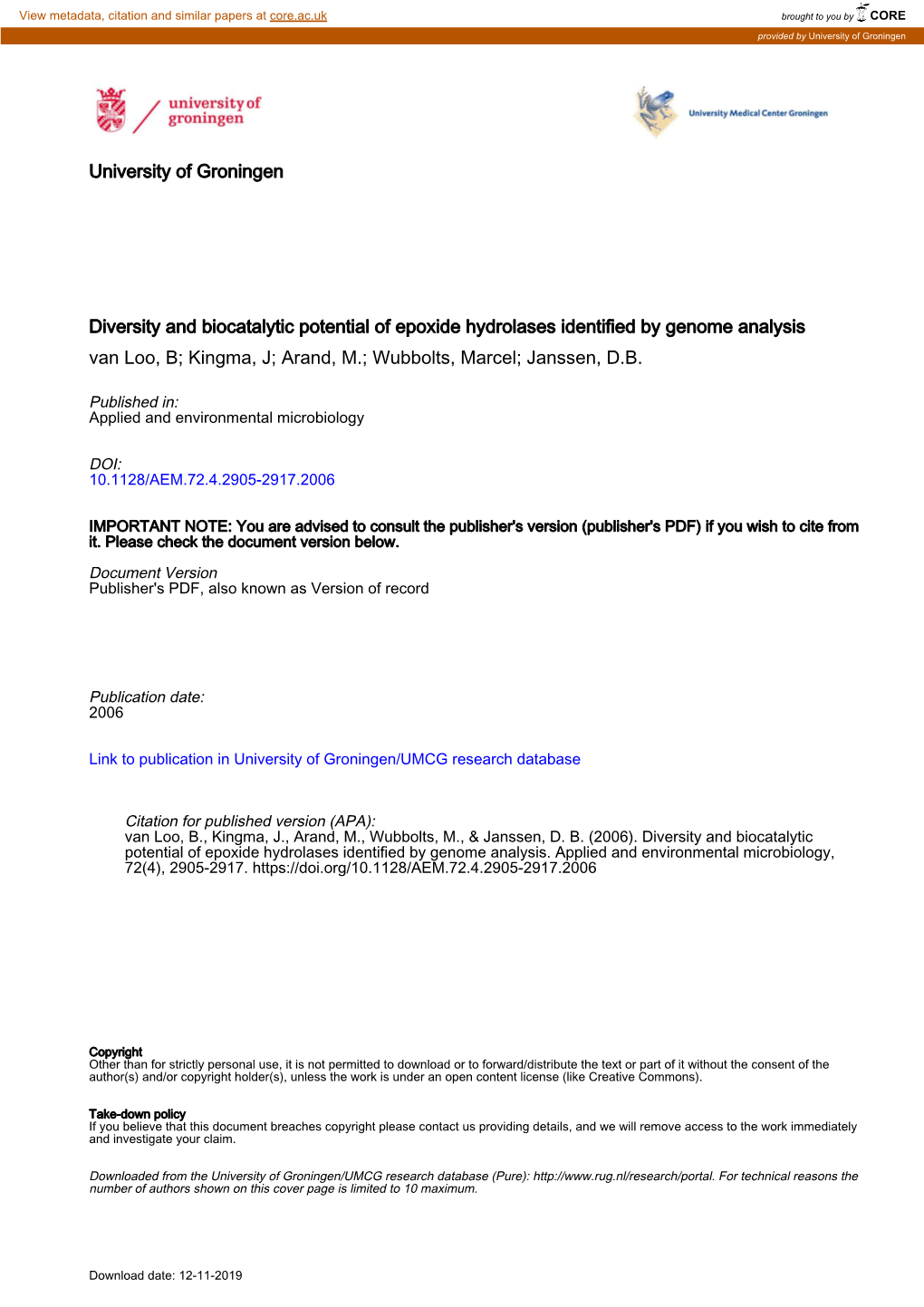
Load more
Recommended publications
-
Diversity and Mechanisms of Bacterial Dehalogenation Reactions High Number of Halogen Substituents
O.B. Janssen, T. Bosma and G.J. Poelarends Diversity and Mechanisms of 8acterial Dehalogenation Reactions Abstract Halogenated aliphatic compounds occur widespread as environmental pollutants. Since many of these compounds are xenobiotics and show large dif ferences in degradability which can be correlated to critical steps in catabolic pathways, they are suitable for studies on the evolution of dehalogenating pathways. We have investigated the degradation of 1,2-dichloroethane and 1,3- dichloropropene in detail. For both compounds, the initial step is hydrolytic dehalogenation. The 1,2-dichloroethane and 1,3-dichloropropene dehalogenases we re found to belong to different groups of identical enzymes detected in bac teria isolated from various sites. Genetic analysis and adaptation experiments indicated th at the 1,2-dichloroethane degradation pathway may be of recent evolutionary origin. The large-scale use of 1,3-dichloropropene in agriculture may have contributed to the distribution of genes encoding hydrolytic dehalogenases in the environment. Introduction The biodegradation of synthetic chlorinated chemicals that enter the environ ment is dependent on the capacity of microbial enzymes to recognize these xenobiotic molecules and cleave or labilize carbon-halogen bonds (Janssen et al., 1994). Microbiological studies have led to the isolation of a range of organisms that degrade halogenated aliphatic compounds and use them as a carbon source for growth, and a several dehalogenating enzymes that directly act on carbon halogen bonds have now been identified (Leisinger and Bader 1993; Janssen et al., 1994; Fetzner and Lingens, 1994). In a few cases, the carbon-halogen bond is not directly cleaved but labilized by introduction of other functional groups (Ensley, 1991). -
Purification and Characterization of a Haloalkane
APPLIED AND ENVIRONMENTAL MICROBIOLOGY, Sept. 1997, p. 3707–3710 Vol. 63, No. 9 0099-2240/97/$04.0010 Copyright © 1997, American Society for Microbiology Purification and Characterization of a Haloalkane Dehalogenase of a New Substrate Class from a g-Hexachlorocyclohexane- Degrading Bacterium, Sphingomonas paucimobilis UT26 YUJI NAGATA,1* KEISUKE MIYAUCHI,1 JIRI DAMBORSKY,2 KATKA MANOVA,2 2 1 ALENA ANSORGOVA, AND MASAMICHI TAKAGI Department of Biotechnology, The University of Tokyo, Yayoi, Bunkyo-ku, Tokyo 113, Japan,1 and Laboratory of Biomolecular Structure and Dynamics, Masaryk University, Kotlarska 2, 611 37 Brno, Czech Republic2 Received 30 December 1996/Accepted 10 June 1997 The linB gene product (LinB), 1,3,4,6-tetrachloro-1,4-cyclohexadiene halidohydrolase, which is involved in the degradation of g-hexachlorocyclohexane in Sphingomonas paucimobilis UT26 (Y. Nagata, T. Nariya, R. Ohtomo, M. Fukuda, K. Yano, and M. Takagi, J. Bacteriol. 175:6403–6410, 1993), was overproduced in E. coli and purified to homogeneity. The molecular mass of LinB was deduced to be 30 kDa by gel filtration chromatography and 32 kDa by electrophoresis on sodium dodecyl sulfate-polyacrylamide gel, indicating that LinB is a monomeric enzyme. The optimal pH for activity was 8.2. Not only monochloroalkanes (C3 to C10) but also dichloroalkanes, bromoalkanes, and chlorinated aliphatic alcohols were good substrates for LinB, sug- gesting that LinB is a haloalkane dehalogenase with a broad range of substrate specificity. These results indicate that LinB shares properties with another haloalkane dehalogenase, DhlA (S. Keuning, D. B. Janssen, and B. Witholt, J. Bacteriol. 163:635–639, 1985), which shows significant similarity to LinB in primary structure (D. -
University of Groningen Bacterial Growth on Halogenated
University of Groningen Bacterial Growth on Halogenated Aliphatic Hydrocarbons Janssen, Dick B.; Oppentocht, Jantien E.; Poelarends, Gerrit J. Published in: EPRINTS-BOOK-TITLE IMPORTANT NOTE: You are advised to consult the publisher's version (publisher's PDF) if you wish to cite from it. Please check the document version below. Document Version Publisher's PDF, also known as Version of record Publication date: 2003 Link to publication in University of Groningen/UMCG research database Citation for published version (APA): Janssen, D. B., Oppentocht, J. E., & Poelarends, G. J. (2003). Bacterial Growth on Halogenated Aliphatic Hydrocarbons: Genetics and Biochemistry. In EPRINTS-BOOK-TITLE Copyright Other than for strictly personal use, it is not permitted to download or to forward/distribute the text or part of it without the consent of the author(s) and/or copyright holder(s), unless the work is under an open content license (like Creative Commons). Take-down policy If you believe that this document breaches copyright please contact us providing details, and we will remove access to the work immediately and investigate your claim. Downloaded from the University of Groningen/UMCG research database (Pure): http://www.rug.nl/research/portal. For technical reasons the number of authors shown on this cover page is limited to 10 maximum. Download date: 12-11-2019 Chapter 7 BACTERIAL GROWTH ON HALOGENATED ALIPHATIC HYDROCARBONS: GENETICS AND BIOCHEMISTRY DICK B. JANSSEN, JANTIEN E. OPPENTOCHT AND GERRIT J. POELARENDS Biochemical Laboratory, Groningen Biomolecular Sciences and Biotechnology Institute, University of Groningen, Groningen, The Netherlands 1. INTRODUCTION Many synthetically produced halogenated aliphatic compounds are xenobiotic chemicals in the sense that they do not naturally occur on earth at biologically significant concentrations. -
Crystal Structure of the Cystic Fibrosis Transmembrane Conductance
JOURNAL OF BACTERIOLOGY, Apr. 2010, p. 1785–1795 Vol. 192, No. 7 0021-9193/10/$12.00 doi:10.1128/JB.01348-09 Copyright © 2010, American Society for Microbiology. All Rights Reserved. Crystal Structure of the Cystic Fibrosis Transmembrane Conductance Regulator Inhibitory Factor Cif Reveals Novel Active-Site Features of an Epoxide Hydrolase Virulence Factorᰔ† Christopher D. Bahl,1 Christophe Morisseau,2 Jennifer M. Bomberger,3 Bruce A. Stanton,3 Bruce D. Hammock,2 George A. O’Toole,4 and Dean R. Madden1* Department of Biochemistry, Dartmouth Medical School, Hanover, New Hampshire 037551; Department of Entomology and Cancer Center, University of California, Davis, California 95616 2; Department of Physiology, Dartmouth Medical School, Hanover, New Hampshire3; and Department of Microbiology and Immunology, Dartmouth Medical School, Hanover, New Hampshire 037554 Received 13 October 2009/Accepted 15 January 2010 Downloaded from Cystic fibrosis transmembrane conductance regulator (CFTR) inhibitory factor (Cif) is a virulence factor secreted by Pseudomonas aeruginosa that reduces the quantity of CFTR in the apical membrane of human airway epithelial cells. Initial sequence analysis suggested that Cif is an epoxide hydrolase (EH), but its sequence violates two strictly conserved EH motifs and also is compatible with other ␣/ hydrolase family members with diverse substrate specificities. To investigate the mechanistic basis of Cif activity, we have determined its structure at 1.8-Å resolution by X-ray crystallography. The catalytic triad consists of residues jb.asm.org Asp129, His297, and Glu153, which are conserved across the family of EHs. At other positions, sequence deviations from canonical EH active-site motifs are stereochemically conservative. -
Analogous Enzymes: Independent Inventions in Enzyme Evolution Michael Y
Downloaded from genome.cshlp.org on September 30, 2021 - Published by Cold Spring Harbor Laboratory Press RESEARCH Analogous Enzymes: Independent Inventions in Enzyme Evolution Michael Y. Galperin,1 D. Roland Walker,1,2 and Eugene V. Koonin1,3 1National Center for Biotechnology Information (NCBI), National Library of Medicine, National Institutes of Health, Bethesda, Maryland 20894 USA; 2Department of Biology, Johns Hopkins University, Baltimore, Maryland 21218 USA It is known that the same reaction may be catalyzed by structurally unrelated enzymes. We performed a systematic search for such analogous (as opposed to homologous) enzymes by evaluating sequence conservation among enzymes with the same enzyme classification (EC) number using sensitive, iterative sequence database search methods. Enzymes without detectable sequence similarity to each other were found for 105 EC numbers (a total of 243 distinct proteins). In 34 cases, independent evolutionary origin of the suspected analogous enzymes was corroborated by showing that they possess different structural folds. Analogous enzymes were found in each class of enzymes, but their overall distribution on the map of biochemical pathways is patchy, suggesting multiple events of gene transfer and selective loss in evolution, rather than acquisition of entire pathways catalyzed by a set of unrelated enzymes. Recruitment of enzymes that catalyze a similar but distinct reaction seems to be a major scenario for the evolution of analogous enzymes, which should be taken into account for functional annotation of genomes. For many analogous enzymes, the bacterial form of the enzyme is different from the eukaryotic one; such enzymes may be promising targets for the development of new antibacterial drugs. -
From Rhizobium Sp. RC1
Adamu et al. SpringerPlus (2016) 5:695 DOI 10.1186/s40064-016-2328-9 REVIEW Open Access L‑2‑Haloacid dehalogenase (DehL) from Rhizobium sp. RC1 Aliyu Adamu1, Roswanira Abdul Wahab2 and Fahrul Huyop1* *Correspondence: [email protected] Abstract 1 Department L-2-Haloacid dehalogenase (DehL) from Rhizobium sp. RC1 is a stereospecific enzyme of Biotechnology and Medical Engineering, that acts exclusively on L-isomers of 2-chloropropionate and dichloroacetate. The Faculty of Biosciences amino acid sequence of this enzyme is substantially different from those of other and Medical Engineering, L-specific dehalogenases produced by other organisms. DehL has not been crystallised, Universiti Teknologi Malaysia, 81310 Johor Baharu, Johor, and hence its three-dimensional structure is unavailable. Herein, we review what is Malaysia known concerning DehL and tentatively identify the amino acid residues important for Full list of author information catalysis based on a comparative structural and sequence analysis with well-character- is available at the end of the article ised L-specific dehalogenases. Keywords: DehL, Rhizobium sp. RC1, Dehalogenation, Catalytic amino acid residues Background Halogenated organic compounds contain at least one carbon–halogen bond. More than 3800 different, naturally occurring, halogenated organic compounds are present in huge amounts in the biosphere (Gribble 2003). However, even more have been industri- ally produced, which is attributable to their diverse use in various industrially related products, e.g., agrochemicals, pharmaceuticals, and solvents (Fetzner and Lingens 1994). These compounds have caused serious environmental pollution owing to their direct toxicity, their potentially toxic breakdown products, and their persistence in the environment. Interestingly, a number of bacteria use halogenated organic compounds as their sole carbon and energy sources, thereby helping to reverse the effects of environmental hal- ogen-associated pollution. -
Designing Novel Biochemical Pathways to Commodity Chemicals Using
bioRxiv preprint doi: https://doi.org/10.1101/2020.12.31.425007; this version posted January 3, 2021. The copyright holder for this preprint (which was not certified by peer review) is the author/funder, who has granted bioRxiv a license to display the preprint in perpetuity. It is made available under aCC-BY-ND 4.0 International license. 1 Designing novel biochemical pathways to commodity chemicals using 2 ReactPRED and RetroPath2.0 3 4 5 6 Authors and Affiliations 7 • Eleanor Vigrass 8 • M. Ahsanul Islam 9 • Department of Chemical Engineering, Loughborough University, Loughborough, 10 Leicestershire, LE11 3TU, UK 11 12 Corresponding Author 13 • M. Ahsanul Islam ([email protected]) 14 15 16 17 18 19 20 21 22 23 24 25 26 1 bioRxiv preprint doi: https://doi.org/10.1101/2020.12.31.425007; this version posted January 3, 2021. The copyright holder for this preprint (which was not certified by peer review) is the author/funder, who has granted bioRxiv a license to display the preprint in perpetuity. It is made available under aCC-BY-ND 4.0 International license. 27 Abstract 28 Commodity chemicals are high-demand chemicals, used by chemical industries to synthesise 29 countless chemical products of daily use. For many of these chemicals, the main production 30 process uses petroleum-based feedstocks. Concerns over these limited resources and their 31 associated environmental problems, as well as mounting global pressure to reduce CO2 32 emissions have motivated efforts to find biochemical pathways capable of producing these 33 chemicals. Advances in metabolic engineering have led to the development of technologies 34 capable of designing novel biochemical pathways to commodity chemicals. -
Madison, WI (US); Paul Otto, Madison, G:25: R 1339: St
USOO842O367B2 (12) United States Patent (10) Patent No.: US 8.420,367 B2 Darzins et al. (45) Date of Patent: Apr. 16, 2013 (54) POLYNUCLEOTIDES ENCODING MUTANT 5,700,935 A 12/1997 Takenishi et al. HYDROLASE PROTEINS WITHENHANCED 3. A 6. 3. E. KINETICS AND FUNCTIONAL EXPRESSION 5,932,421war. A 8/1999 Ginsberga ca. et al. 5,945,526 A 8, 1999 Lee et al. (75) Inventors: Aldis Darzins, Highlands Ranch, CO 6.255.461 B1 7/2001 Mosbach et al. (US); Lance P. Encell, Fitchburg, WI 6,333,154 B1 12/2001 Ladant et al. (US); Rachel Friedman Ohana, 6,416,733 B1 7/2002 Barrett et al. Madison, WI (US); Paul Otto, Madison, g:25: R 1339: St. et al. WI (US); Gediminas Vidugiris, 6,800.453 B2 102004 Labaer et al. Fitchburg, WI (US); Keith V. Wood, Mt. 7,078,504 B2 7/2006 Short et al. Horeb, WI (US); Monika G. Wood, Mt. 7,238,842 B2 7/2007 Wood et al. Horeb, WI (US); Kris Zimmerman, 7,425,436 B2 9, 2008 Darzins et al. Madison, WI (US); Michael R. Slater, E. R. E. E." Madison, WI (US); Kate Qin Zhao, 2002.01371.71 A1 9, 2002 Short et al. Verona, WI (US) 2003/O166957 A1 9, 2003 Benneteau et al. 2004/O152880 A1 8, 2004 Minden (73) Assignee: Promega Corporation, Madison, WI 2005/0048580 A1 3/2005 Labaer et al. (US) 2005/OO95651 A1 5/2005 Camarero et al. 2006, OO24808 A1 2/2006 Darzins et al. 2007/0O874OO A1 4/2007 Darzins et al. -
A Review on Non-Stereospecific Haloalkanoic Acid Dehalogenases
African Journal of Biotechnology Vol. 10(48), pp. 9725-9736, 29 August, 2011 Available online at http://www.academicjournals.org/AJB DOI: 10.5897/AJB11.934 ISSN 1684–5315 © 2011 Academic Journals Review A review on non-stereospecific haloalkanoic acid dehalogenases Tengku Haziyamin Tengku Abdul Hamid 1, Azzmer Azzar Abdul Hamid 1 and Fahrul Huyop 2* 1Department of Biotechnology, Faculty of Science, International Islamic University, Bandar Indera Mahkota, 25200 Kuantan, Pahang, Malaysia. 2Department of Industrial Biotechnology, Faculty of Biosciences and Bioengineering, Universiti Teknologi Malaysia, 81300 Skudai, Johor, Malaysia. Accepted 1 July, 2011 Haloalkanoic acid dehalogenases remove halides from organic haloacids and have potential as bioremediation agents. DehE from Rhizobium sp . RC1, DehI from Pseudomonas putida PP3 and D,L- DEX 113 from Pseudomonas sp. 113 are non-stereospecific dehalogenases that invert the configurations of D- and L- carbons bound to a halogen. The kinetics of DehE has been partially characterized and brominated compounds have greater specificity constant values than do the corresponding chlorinated compounds. The sequence of DehE is similar to that of DehI; therefore, the two enzymes may have similar structures and functions. The three-dimensional structure of DehI is known and its reaction mechanism was inferred from its structure and a mutagenesis study of D,L-DEX 113. Aspartate residues at positions 189 and 194 in DehI and D,L-DEX 113 were predicted to be involved in catalysis. These residues activate a water molecule that directly attacks the chiral carbon. Because DehE and DehI are sequentially related, delineating the structure of DehE is important to ascertain if the catalytic residues and reaction mechanism are the same for both enzymes. -
Hydrolytic Defluorination • Haloacetate Dehalogenase (EC 3.8.1.3) • Aerobic Bacteria: Pseudomonas Spp., Moraxella Sp
Bioremediation of PFAS: Promise and Challenges Yujie Men Ph.D., Assistant Professor December 4, 2020 #SerdpEstcp2020 Outline Part 1: History of microbial cleavage of C–F in organofluorines Part 2: Current research status on PFAS biotransformation Part 3: Implications in biotechnologies for PFAS remediation 2 #SerdpEstcp2020 Part 1: Microbial cleavage of C–F in organofluorines C−F bond and bioavailability • C–F bond: the strongest single bond in nature Bond kJ/mol Bond kJ/mol C‒F 439 C‒C 347 C‒H 414 C‒Cl 331 C‒O 351 C‒N 293 • Microbial cleavage of C–F: thermodynamically feasible, kinetically hindered ∆G0 (kJ/mol) Reaction Defluorination Dechlorination Tetrahalomethane → Trihalomethane + H+ + halide‒ -89 -188 (Dolfing 2003; Parsons et al., 2008) 3 #SerdpEstcp2020 Part 1: Microbial cleavage of C–F in organofluorines Hydrolytic defluorination • Haloacetate dehalogenase (EC 3.8.1.3) • Aerobic bacteria: Pseudomonas spp., Moraxella sp. B, Burkholderia sp. FA1, Aureobacterium sp. strain RH025 • Substrate: monofluoroacetate • No reports on PFAS as substrates - + O O O O O H2O F + H O Aureobacterium sp. F - HO - Strain RH025 O Haloacetate O dehalogenase H O + F Monofluoroacetate Glycolate F 2 H OH (Goldman, 1965&1969; Key et al., 1997; Natarajan et al., 2005; Kurihara et al., 2008) 4 #SerdpEstcp2020 Part 1: Microbial C–F bond cleavage in organofluorines Reductive defluorination • Unknown enzyme(s) • Anaerobic, methanogenic communities • Poor reproducibility • Cometabolism • No follow-up reports since 2000 O O O O F - F - F - - O O O H3C O F F F Trifluoroacetate Difluoroacetate Monofluoroacetate Acetate (Visscher et al., 1994; Key et al., 1997; Kim et al., 2000) 5 #SerdpEstcp2020 Part 1: Microbial C–F bond cleavage in organofluorines Reductive defluorination (cont’d) • Pyruvate dehydrogenase from E. -
Enzymatic Defluorination of Fluorinated Compounds
Seong et al. Appl Biol Chem (2019) 62:62 https://doi.org/10.1186/s13765-019-0469-6 INVITED REVIEW Open Access Enzymatic defuorination of fuorinated compounds Hyeon Jeong Seong†, Seong Woo Kwon†, Dong‑Cheol Seo, Jin‑Hyo Kim* and Yu‑Sin Jang* Abstract Fluorine‑containing compounds are widely used because they have properties required in textiles and coatings for electronic, automotive, and outdoor products. However, fuorinated compounds do not easily break down in nature, which has resulted in their accumulation in the environment as well as the human body. Recently, the enzymatic defuorination of fuorine‑containing compounds has gained increasing attention. Here, we review the enzymatic defuorination reactions of fuorinated compounds. Furthermore, we review the enzyme engineering strategies for cleaving C–F bonds, which have the highest dissociation energy found in organic compounds. Keywords: C–F bond, Defuorination, Fluorine, Perfuorinated compound Introduction and the corresponding enzymes, have not been well Because perfuorinated compounds (PFCs) repel both elucidated. water and oil, they are used as durable repellent treat- On the other hand, fuorinated compounds such as ments for textiles such as outdoor clothes and for home fuoroacetate and 5′-fuoro-5′-deoxyadenosine have been products such as carpets [1]. In addition, PFCs are widely identifed as natural products in nature [10, 11], dem- used in the production of fuoropolymers such as polyte- onstrating the existence of a biosynthesis pathway for trafuoroethylene (trade name, Tefon), which is widely fuorinated compounds in organisms [12]. A representa- used in coatings for electronic, automotive, and outdoor tive pathway of this type is the C–F bond forming reac- products [2]. -
Canada Archives Canada Published Heritage Direction Du Branch Patrimoine De I'edition
DISCOVERY AND CHARACTERIZATION OF HYDROLYTIC DEHALOGENASES FROM GENOMIC DATA by Max Wong A thesis submitted in conformity with the requirements for the degree of Master of Applied Science Graduate Department of Chemical Engineering and Applied Chemistry University of Toronto Copyright Q 2008 by Max Wong Library and Bibliotheque et 1*1 Archives Canada Archives Canada Published Heritage Direction du Branch Patrimoine de I'edition 395 Wellington Street 395, rue Wellington Ottawa ON K1A0N4 Ottawa ON K1A0N4 Canada Canada Your file Votre reference ISBN: 978-0-494-38866-2 Our file Notre reference ISBN: 978-0-494-38866-2 NOTICE: AVIS: The author has granted a non L'auteur a accorde une licence non exclusive exclusive license allowing Library permettant a la Bibliotheque et Archives and Archives Canada to reproduce, Canada de reproduire, publier, archiver, publish, archive, preserve, conserve, sauvegarder, conserver, transmettre au public communicate to the public by par telecommunication ou par I'lnternet, prefer, telecommunication or on the Internet, distribuer et vendre des theses partout dans loan, distribute and sell theses le monde, a des fins commerciales ou autres, worldwide, for commercial or non sur support microforme, papier, electronique commercial purposes, in microform, et/ou autres formats. paper, electronic and/or any other formats. The author retains copyright L'auteur conserve la propriete du droit d'auteur ownership and moral rights in et des droits moraux qui protege cette these. this thesis. Neither the thesis Ni la these ni des extraits substantiels de nor substantial extracts from it celle-ci ne doivent etre imprimes ou autrement may be printed or otherwise reproduits sans son autorisation.