Model-Based Schedulability Analysis of Hard Real-Time Java Programs Using Software Transactional Memory
Total Page:16
File Type:pdf, Size:1020Kb
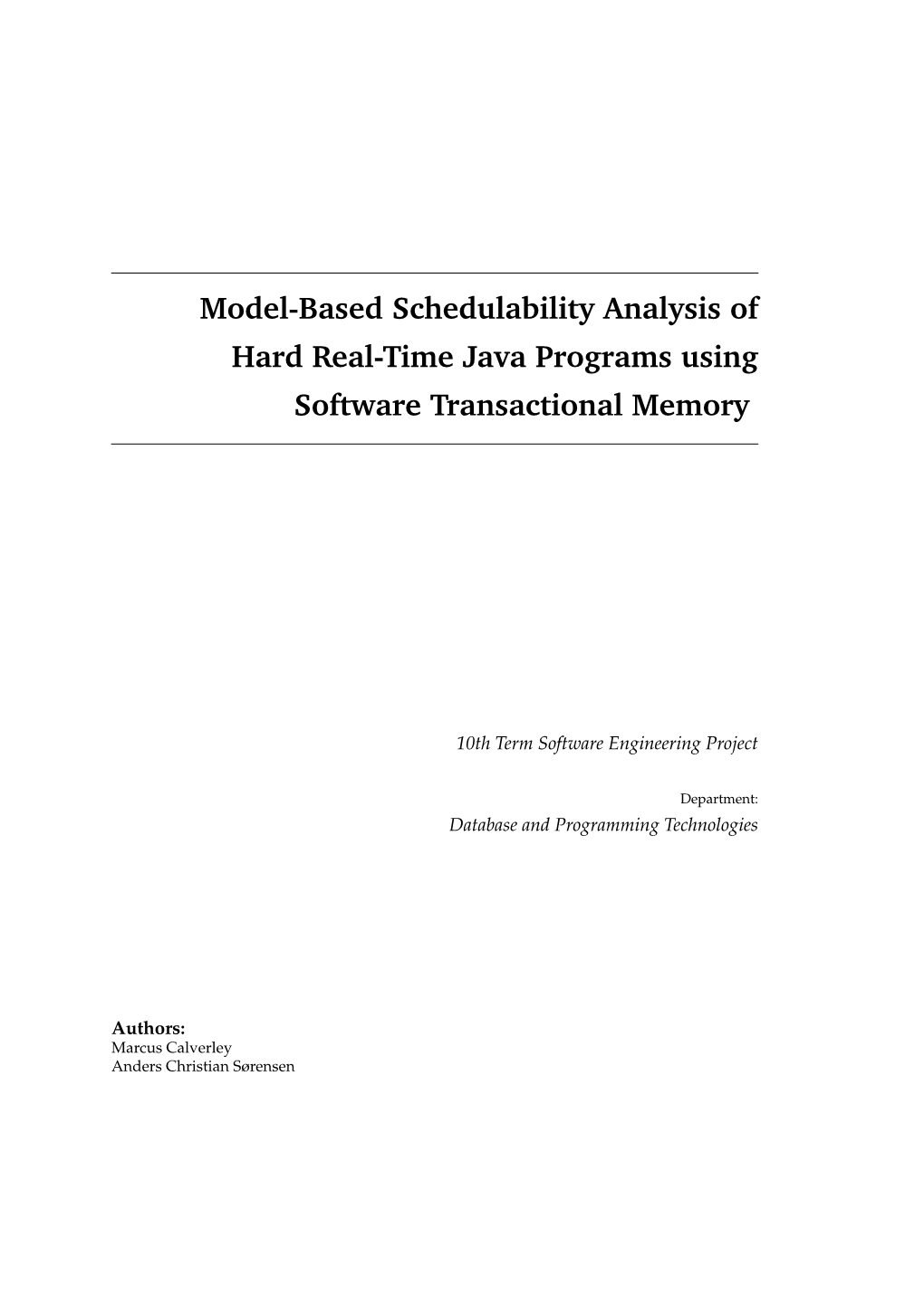
Load more
Recommended publications
-
WCET Analysis of Java Bytecode Featuring Common Execution Environments
WCET Analysis of Java Bytecode Featuring Common Execution Environments Christian Frost, Casper Svenning Jensen, Kasper Søe Luckow, Bent Thomsen Department of Computer Science, Aalborg University Selma Lagerlöfs Vej 300 DK-9220, Aalborg East, Denmark {chrfrost,semadk,luckow,bt}@cs.aau.dk ABSTRACT 1. INTRODUCTION We present a novel tool for statically determining the Worst Java in its traditional form, has inherent issues that makes Case Execution Time (WCET) of Java Bytecode-based pro- it less suitable for development of hard real-time embedded grams called Tool for Execution Time Analysis of Java byte- systems such as the lack of the notion of a deadline and code (TetaJ). This tool differentiates itself from existing high-resolution real-time clocks. To accommodate these, a tools by separating the individual constituents of the execu- variety of initiatives such as the Real-Time Specification tion environment into independent components. The prime for Java (RTSJ) [23] and related profiles: Safety Critical benefit is that it can be used for execution environments Java (SCJ) [16] and Predictable Java (PJ) [9] have been featuring common embedded processors and software im- initiated. These specify certain alterations to the under- plementations of the JVM. TetaJ employs a model checking lying Java Virtual Machine (JVM) and various extensions approach for statically determining WCET where the Java through libraries that, when adhered to, creates a program- program, the JVM, and the hardware are modelled as Net- ming model that can be used for real-time systems devel- works of Timed Automata (NTA) and given as input to the opment in Java. -
Design Decisions for a Java Processor
Design Decisions for a Java Processor Martin Schoeberl JOP.design Strausseng. 2-10/2/55, A-1050 Vienna, AUSTRIA [email protected] Abstract The paper is organized as follows: Section 2 gives an overview of the architecture of JOP. In section 3 some This paper describes design decisions for JOP, a Java design decisions for the implementation in an FPGA are Optimized Processor, implemented in an FPGA. FPGA described. One example of the flexibility of an FPGA density-price relationship makes it now possible to con- based architecture is given in section 4. sider them not only for prototyping of processor designs but also as final implementation technology. However, using an FPGA as target platform for a processor dif- 2 Overview of JOP ferent constraints influence the CPU architecture. Digi- tal building blocks that map well in an ASIC can result Figure 1 shows the datapath of JOP. In the first pipe- in poor resource usage in an FPGA. Considering these line stage Java bytecodes, the instructions of the JVM, constraints in the architecture can result in a tiny soft- are fetched. These bytecodes are translated to addresses core processor. in the micro code. Bytecode branches are also decoded and executed in this stage. A fetched bytecode results in an absolute jump in the micro code (the second stage). If 1 Introduction the bytecode has a 1 to 1 mapping with a JOP instruc- tion, a new bytecode is fetched, resulting in a jump in Common design praxis for embedded systems is us- the micro code in the next cycle. -
JOP: a Java Optimized Processor
JOP: A Java Optimized Processor Martin Schoeberl JOP.design, Strausseng. 2-10/2/55, A-1050 Vienna, AUSTRIA [email protected] Abstract. Java is still not a common language for embedded systems. It posses language features, like thread support, that can improve embedded system de- velopment, but common implementations as interpreter or just-in-time compiler are not practical. JOP is a hardware implementation of the Java Virtual Ma- chine with focus on real-time applications. This paper describes the architecture of JOP and proposes a simple real-time extension of Java for JOP. First applica- tion in an industrial system showed that JOP is one way to use Java in the em- bedded world. 1 Introduction Current software design practice for embedded systems is still archaic compared to software development for desktop systems. C and even Assembler is used on top of a small RTOS. The variety of embedded operating systems is large and this fragmenta- tion of the market leads to high cost. Java [1] can be a way out of this dilemma and possess language features not found in C: - Object-oriented - Memory management with a garbage collector - Implicit memory protection - Threads Memory management and threads are (besides device drivers) the main compo- nents of embedded operating systems. Finding these features in the language embed- ded systems can be programmed in Java without the need of an operating system. Java on desktop systems comes with a large library. However, if Java is stripped down to the core components it has a very small memory footprint. With careful pro- gramming (like using only immortal memory as in [2]) the garbage collector can be avoided. -
A Hardware Abstraction Layer in Java
A Hardware Abstraction Layer in Java MARTIN SCHOEBERL Vienna University of Technology, Austria STEPHAN KORSHOLM Aalborg University, Denmark TOMAS KALIBERA Purdue University, USA and ANDERS P. RAVN Aalborg University, Denmark Embedded systems use specialized I/O devices to interact with their environment, and since they have to be de- pendable, it is attractive to use a modern, type-safe programming language like Java to develop programs for them. However, standard Java, as a platform independent language, delegates access to I/O devices, direct mem- ory access and interrupt handling to some underlying operating system or kernel, but in the embedded systems domain resources are scarce and a Java virtual machine (JVM) without an underlying middleware is an attractive architecture. The contribution of this paper is a proposal for Java packages with hardware objects and interrupt handlers that interfaces to such a JVM. We provide implementations of the proposal directly in hardware, directly as extensions of standard interpreters, and finally with an operating system middleware. The latter solution is mainly seen as a migration path allowing Java programs to coexist with legacy system components. An important aspect of the proposal is that it is compatible with the Real-Time Specification for Java (RTSJ). Categories and Subject Descriptors: D.4.7 [Operating Systems]: Organization and Design—Real-time sys- tems and embedded systems; D.3.3 [Programming Languages]: Language Classifications—Object-oriented languages; D.3.3 [Programming Languages]: Language Constructs and Features—Input/output General Terms: Languages, Design, Implementation Additional Key Words and Phrases: Device driver, embedded system, Java, Java virtual machine 1. -
Application Experiences with a Real-Time Java Processor
Proceedings of the 17th World Congress The International Federation of Automatic Control Seoul, Korea, July 6-11, 2008 Application Experiences with a Real-Time Java Processor Martin Schoeberl ∗ ∗ Institute of Computer Engineering, Vienna University of Technology, Austria (e-mail: [email protected]). Abstract: In this paper we present three different industrial real-time applications that are based on an embedded Java processor. Although from different application domains all three projects have one topic in common: communication. Today’s embedded systems are networked systems. Either a proprietary protocol is used due to legacy applications or for real-time aspects or standard Internet protocols are used. We present the challenges and solutions for this variety of protocols in small, memory constraint embedded devices. 1. INTRODUCTION JOP is implemented in a field-programmable gate array (FPGA). The logic resource consumption is configurable Embedded systems in the control and automation domain in the range of 2000–3000 logic cells (LC). That size is 1/3 become more and more complex. In the desktop and of the soft-core RISC processor LEON, see Gaisler (2002), server domain the increasing complexity of systems can that is used by ESA for space missions. be handled by advanced programming languages. Java has Implementation of a processor in an FPGA is a little bit been seen as a very productive object-oriented language. more expensive than using an ASIC processor. However, In this paper we show that Java can also be used in the additional application logic, such as a communication embedded domain. We present three real-world embedded controller or an AD converter, can also be integrated applications written entirely in Java. -
Extending Java for Heterogeneous Embedded System Description
Extending Java for Heterogeneous Embedded System Description Gary Plumbridge Neil Audsley Department of Computer Science Department of Computer Science University of York, York, UK, YO10 5GH University of York, York, UK, YO10 5GH [email protected] [email protected] Abstract—This paper introduces Machine Java, a framework • Mapping of required functionality onto available hard- of classes for the Java programming language that enable the ware components must be done by hand. description of software for systems with heterogeneous processing elements (such as CPUs, microcontrollers and function acceler- In this paper we present a small set of class library exten- ators). Intended for the behavioural description of embedded sions to the Java[12] programming language to facilitate the systems, Machine Java encapsulates both the data and control description and implementation of heterogeneous embedded aspects of computation into ‘machine’ objects that are appropri- systems in event driven style. Our primary addition to Java is ate for mapping onto architecturally diverse multiprocessors. a class to represent independent execution and storage, referred System descriptions in Machine Java avoid the need for a separate programming language for each processing element, and to as a Machine. Each machine embodies a computationally makes explicit description of communications between processors discrete element that can operate concurrently with every other unnecessary. Suitability for a wide variety of hardware platforms machine in a system. A major distinction between machines is enhanced by avoiding dependence on notions of shared memory and ordinary threads in Java is that machines may not share or shared timing resources. any memory with one another and interaction is restricted to well defined channels. -
JOP: a Java Optimized Processor for Embedded Real-Time Systems
Downloaded from orbit.dtu.dk on: Oct 04, 2021 JOP: A Java Optimized Processor for Embedded Real-Time Systems Schoeberl, Martin Publication date: 2005 Document Version Publisher's PDF, also known as Version of record Link back to DTU Orbit Citation (APA): Schoeberl, M. (2005). JOP: A Java Optimized Processor for Embedded Real-Time Systems. Vienna University of Technology. http://www.jopdesign.com/ General rights Copyright and moral rights for the publications made accessible in the public portal are retained by the authors and/or other copyright owners and it is a condition of accessing publications that users recognise and abide by the legal requirements associated with these rights. Users may download and print one copy of any publication from the public portal for the purpose of private study or research. You may not further distribute the material or use it for any profit-making activity or commercial gain You may freely distribute the URL identifying the publication in the public portal If you believe that this document breaches copyright please contact us providing details, and we will remove access to the work immediately and investigate your claim. DISSERTATION JOP: A Java Optimized Processor for Embedded Real-Time Systems ausgef¨uhrt zum Zwecke der Erlangung des akademischen Grades eines Doktors der technischen Wissenschaften unter der Leitung von AO.UNIV.PROF. DIPL.-ING. DR.TECHN. ANDREAS STEININGER und AO.UNIV.PROF. DIPL.-ING. DR.TECHN. PETER PUSCHNER Inst.-Nr. E182 Institut f¨ur Technische Informatik eingereicht an der Technischen Universit¨at Wien Fakult¨at f¨ur Informatik von DIPL.-ING. MARTIN SCHOBERL¨ Matr.-Nr. -
Evaluation of a Java Processor
Downloaded from orbit.dtu.dk on: Sep 27, 2021 Evaluation of a Java Processor Schoeberl, Martin Published in: Tagungsband Austrochip 2005 Publication date: 2005 Document Version Early version, also known as pre-print Link back to DTU Orbit Citation (APA): Schoeberl, M. (2005). Evaluation of a Java Processor. In Tagungsband Austrochip 2005 (pp. 127-134) General rights Copyright and moral rights for the publications made accessible in the public portal are retained by the authors and/or other copyright owners and it is a condition of accessing publications that users recognise and abide by the legal requirements associated with these rights. Users may download and print one copy of any publication from the public portal for the purpose of private study or research. You may not further distribute the material or use it for any profit-making activity or commercial gain You may freely distribute the URL identifying the publication in the public portal If you believe that this document breaches copyright please contact us providing details, and we will remove access to the work immediately and investigate your claim. Evaluation of a Java Processor Martin Schoeberl Institute of Computer Engineering Vienna University of Technology, Austria [email protected] TABLE I Abstract— In this paper, we will present the evaluation results for a Java processor, with respect to size and performance. The JOP AND VARIOUS JAVA PROCESSORS Java Optimized Processor (JOP) is an implementation of the Java virtual machine (JVM) in a low-cost FPGA. JOP is the smallest Target Size Speed hardware realization of the JVM available to date. -
A Java Processor Architecture for Embedded Real-Time Systems
Downloaded from orbit.dtu.dk on: Oct 01, 2021 A Java Processor Architecture for Embedded Real-Time Systems Schoeberl, Martin Published in: Journal of Systems Architecture Link to article, DOI: 10.1016/j.sysarc.2007.06.001 Publication date: 2008 Document Version Early version, also known as pre-print Link back to DTU Orbit Citation (APA): Schoeberl, M. (2008). A Java Processor Architecture for Embedded Real-Time Systems. Journal of Systems Architecture, 54(1-2), 265-286. https://doi.org/10.1016/j.sysarc.2007.06.001 General rights Copyright and moral rights for the publications made accessible in the public portal are retained by the authors and/or other copyright owners and it is a condition of accessing publications that users recognise and abide by the legal requirements associated with these rights. Users may download and print one copy of any publication from the public portal for the purpose of private study or research. You may not further distribute the material or use it for any profit-making activity or commercial gain You may freely distribute the URL identifying the publication in the public portal If you believe that this document breaches copyright please contact us providing details, and we will remove access to the work immediately and investigate your claim. A Java Processor Architecture for Embedded Real-Time Systems Martin Schoeberl Institute of Computer Engineering, Vienna University of Technology, Austria Abstract Architectural advancements in modern processor designs increase average performance with features such as pipelines, caches, branch prediction, and out-of-order execution. However, these features complicate worst-case execution time analysis and lead to very conservative estimates. -
JOP Reference Handbook: Building Embedded Systems with a Java Processor
Downloaded from orbit.dtu.dk on: Sep 30, 2021 JOP Reference Handbook: Building Embedded Systems with a Java Processor Schoeberl, Martin Publication date: 2009 Document Version Publisher's PDF, also known as Version of record Link back to DTU Orbit Citation (APA): Schoeberl, M. (2009). JOP Reference Handbook: Building Embedded Systems with a Java Processor. http://www.jopdesign.com/ General rights Copyright and moral rights for the publications made accessible in the public portal are retained by the authors and/or other copyright owners and it is a condition of accessing publications that users recognise and abide by the legal requirements associated with these rights. Users may download and print one copy of any publication from the public portal for the purpose of private study or research. You may not further distribute the material or use it for any profit-making activity or commercial gain You may freely distribute the URL identifying the publication in the public portal If you believe that this document breaches copyright please contact us providing details, and we will remove access to the work immediately and investigate your claim. JOP Reference Handbook JOP Reference Handbook Building Embedded Systems with a Java Processor Martin Schoeberl Copyright c 2009 Martin Schoeberl Martin Schoeberl Strausseng. 2-10/2/55 A-1050 Vienna, Austria Email: [email protected] Visit the accompanying web site on http://www.jopdesign.com/ and the JOP Wiki at http://www.jopwiki.com/ Published 2009 by CreateSpace, http://www.createspace.com/ All rights reserved. No part of this publication may be reproduced, stored in a retrieval system, or transmitted in any form or by any means, electronic, mechanical, recording or otherwise, without the prior written permission of Martin Schoeberl. -
Design Decisions for a Java Processor
Design Decisions for a Java Processor Martin Schoeberl JOP.design Strausseng. 2-10/2/55, A-1050 Vienna, AUSTRIA [email protected] Abstract The paper is organized as follows: Section 2 gives an overview of the architecture of JOP. In section 3 some This paper describes design decisions for JOP, a Java design decisions for the implementation in an FPGA are Optimized Processor, implemented in an FPGA. FPGA described. One example of the flexibility of an FPGA density-price relationship makes it now possible to con- based architecture is given in section 4. sider them not only for prototyping of processor designs but also as final implementation technology. However, using an FPGA as target platform for a processor dif- 2 Overview of JOP ferent constraints influence the CPU architecture. Digi- tal building blocks that map well in an ASIC can result Figure 1 shows the datapath of JOP. In the first pipe- in poor resource usage in an FPGA. Considering these line stage Java bytecodes, the instructions of the JVM, constraints in the architecture can result in a tiny soft- are fetched. These bytecodes are translated to addresses core processor. in the micro code. Bytecode branches are also decoded and executed in this stage. A fetched bytecode results in an absolute jump in the micro code (the second stage). If 1 Introduction the bytecode has a 1 to 1 mapping with a JOP instruc- tion, a new bytecode is fetched, resulting in a jump in Common design praxis for embedded systems is us- the micro code in the next cycle. -
Time-Predictable Stack Caching
Downloaded from orbit.dtu.dk on: Dec 21, 2017 Time-predictable Stack Caching Abbaspourseyedi, Sahar; Schoeberl, Martin; Sparsø, Jens Publication date: 2016 Document Version Publisher's PDF, also known as Version of record Link back to DTU Orbit Citation (APA): Abbaspourseyedi, S., Schoeberl, M., & Sparsø, J. (2016). Time-predictable Stack Caching. Kgs. Lyngby: Technical University of Denmark (DTU). (DTU Compute PHD-2015; No. 385). General rights Copyright and moral rights for the publications made accessible in the public portal are retained by the authors and/or other copyright owners and it is a condition of accessing publications that users recognise and abide by the legal requirements associated with these rights. • Users may download and print one copy of any publication from the public portal for the purpose of private study or research. • You may not further distribute the material or use it for any profit-making activity or commercial gain • You may freely distribute the URL identifying the publication in the public portal If you believe that this document breaches copyright please contact us providing details, and we will remove access to the work immediately and investigate your claim. Time-predictable Stack Caching Sahar Abbaspourseyedi Kongens Lyngby 2015 IMM-PhD-2015-385 Technical University of Denmark Department of Applied Mathematics and Computer Science Matematiktorvet, building 303B, 2800 Kongens Lyngby, Denmark Phone +45 4525 3351 [email protected] www.compute.dtu.dk IMM-PhD-2015-385 Summary (English) Embedded systems are computing systems for controlling and interacting with physical environments. Embedded systems with special timing constraints where the system needs to meet deadlines are referred to as real-time systems.