Comparative Genomics of the Major Parasitic Worms
Total Page:16
File Type:pdf, Size:1020Kb
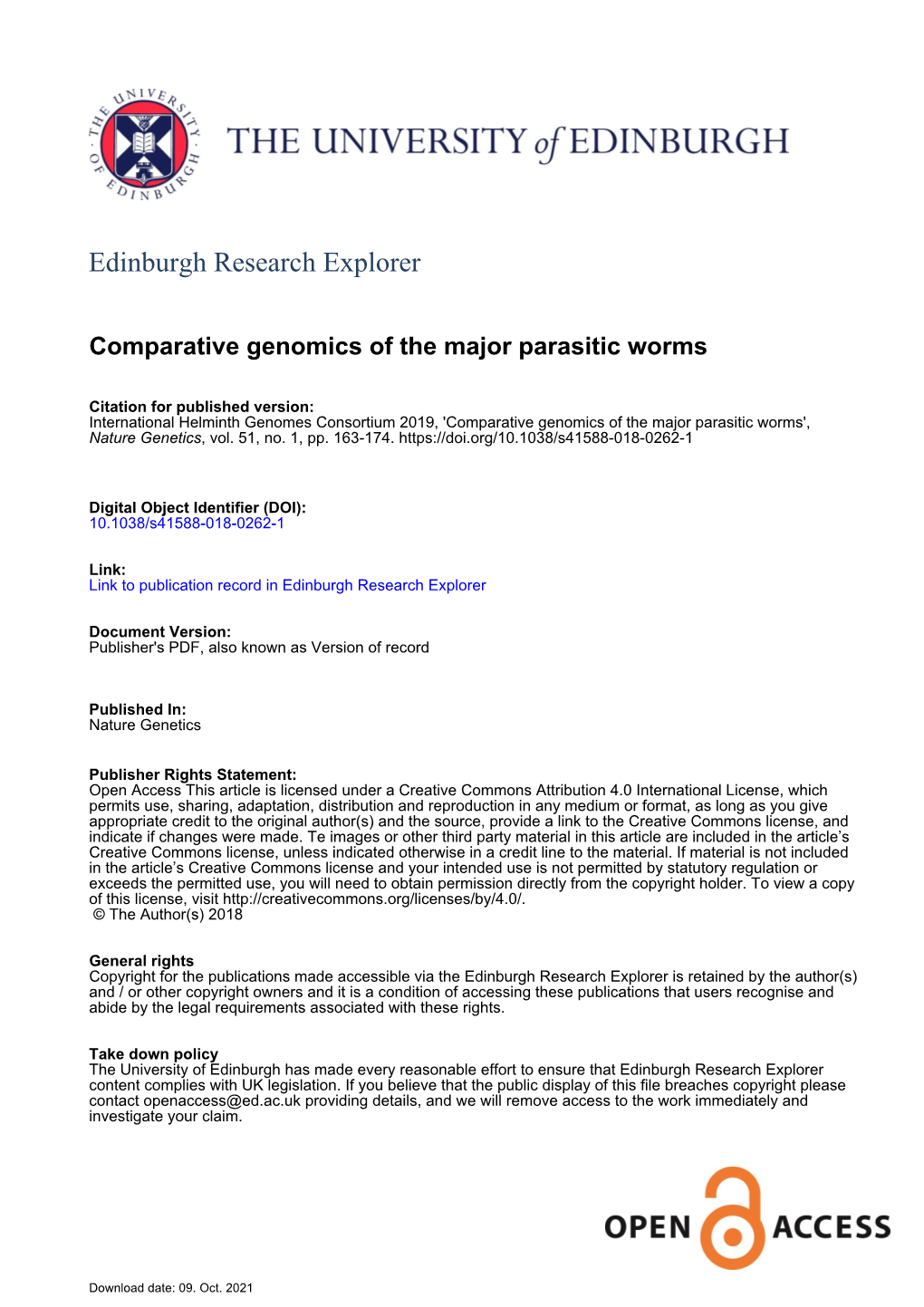
Load more
Recommended publications
-
Toxocariasis: a Rare Cause of Multiple Cerebral Infarction Hyun Hee Kwon Department of Internal Medicine, Daegu Catholic University Medical Center, Daegu, Korea
Case Report Infection & http://dx.doi.org/10.3947/ic.2015.47.2.137 Infect Chemother 2015;47(2):137-141 Chemotherapy ISSN 2093-2340 (Print) · ISSN 2092-6448 (Online) Toxocariasis: A Rare Cause of Multiple Cerebral Infarction Hyun Hee Kwon Department of Internal Medicine, Daegu Catholic University Medical Center, Daegu, Korea Toxocariasis is a parasitic infection caused by the roundworms Toxocara canis or Toxocara cati, mostly due to accidental in- gestion of embryonated eggs. Clinical manifestations vary and are classified as visceral larva migrans or ocular larva migrans according to the organs affected. Central nervous system involvement is an unusual complication. Here, we report a case of multiple cerebral infarction and concurrent multi-organ involvement due to T. canis infestation of a previous healthy 39-year- old male who was admitted for right leg weakness. After treatment with albendazole, the patient’s clinical and laboratory results improved markedly. Key Words: Toxocara canis; Cerebral infarction; Larva migrans, visceral Introduction commonly involved organs [4]. Central nervous system (CNS) involvement is relatively rare in toxocariasis, especially CNS Toxocariasis is a parasitic infection caused by infection with presenting as multiple cerebral infarction. We report a case of the roundworm species Toxocara canis or less frequently multiple cerebral infarction with lung and liver involvement Toxocara cati whose hosts are dogs and cats, respectively [1]. due to T. canis infection in a previously healthy patient who Humans become infected accidentally by ingestion of embry- was admitted for right leg weakness. onated eggs from contaminated soil or dirty hands, or by in- gestion of raw organs containing encapsulated larvae [2]. -
Gastrointestinal Helminthic Parasites of Habituated Wild Chimpanzees
Aus dem Institut für Parasitologie und Tropenveterinärmedizin des Fachbereichs Veterinärmedizin der Freien Universität Berlin Gastrointestinal helminthic parasites of habituated wild chimpanzees (Pan troglodytes verus) in the Taï NP, Côte d’Ivoire − including characterization of cultured helminth developmental stages using genetic markers Inaugural-Dissertation zur Erlangung des Grades eines Doktors der Veterinärmedizin an der Freien Universität Berlin vorgelegt von Sonja Metzger Tierärztin aus München Berlin 2014 Journal-Nr.: 3727 Gedruckt mit Genehmigung des Fachbereichs Veterinärmedizin der Freien Universität Berlin Dekan: Univ.-Prof. Dr. Jürgen Zentek Erster Gutachter: Univ.-Prof. Dr. Georg von Samson-Himmelstjerna Zweiter Gutachter: Univ.-Prof. Dr. Heribert Hofer Dritter Gutachter: Univ.-Prof. Dr. Achim Gruber Deskriptoren (nach CAB-Thesaurus): chimpanzees, helminths, host parasite relationships, fecal examination, characterization, developmental stages, ribosomal RNA, mitochondrial DNA Tag der Promotion: 10.06.2015 Contents I INTRODUCTION ---------------------------------------------------- 1- 4 I.1 Background 1- 3 I.2 Study objectives 4 II LITERATURE OVERVIEW --------------------------------------- 5- 37 II.1 Taï National Park 5- 7 II.1.1 Location and climate 5- 6 II.1.2 Vegetation and fauna 6 II.1.3 Human pressure and impact on the park 7 II.2 Chimpanzees 7- 12 II.2.1 Status 7 II.2.2 Group sizes and composition 7- 9 II.2.3 Territories and ranging behavior 9 II.2.4 Diet and hunting behavior 9- 10 II.2.5 Contact with humans 10 II.2.6 -
Phylum: Nematoda Basic Features
Lec: 1 Nematodes 3rd class Dr.Omaima I.M. Phylum: Nematoda Basic Features: Roundworms get their name from their round cross section Long thread-like bodies Usually very small to microscopic, some parasitic members however may be a metre long Simple tube-like gut with a mouth and anus No circulatory system, gas exchange and excretion are by diffusion across the body wall There is very little superficial difference between nematode species, they all look pretty much like larger or smaller, somewhat fatter or skinnier versions of each other Sexual reproduction, sexes separate, no asexual reproduction. Males are usually smaller than the females, the females of some species can deposit over 100,000 eggs per day. Basic Nematode Life Cycle Despite the diversity and complexity of many nematode life cycles, all of them can be related to the same basic pattern. This pattern is illustrated by the adjacent figure and consists of two phases, parasitic and pre-parasitic. The parasitic phase takes place inside the definitive host while the pre-parasitic phase occurs either as a freeliving phase in the external environment or inside a second host, called an intermediate host. This basic life cycle also consists of seven stages, an egg, four larval stages (L2, L2, L3, L4) and two adult stages comprising separate males and females. Family : Ascaridae Parascaris equorum found in the small intestine of equids, especially <2 year olds. Main properties Males can reach up to 28 cm length, females up to 50 cm. They have a whitish color and a translucent aspect, and look very much like cooked spaghetti. -
Conservation and Diversification of Small RNA Pathways Within Flatworms Santiago Fontenla1, Gabriel Rinaldi2, Pablo Smircich1,3 and Jose F
Fontenla et al. BMC Evolutionary Biology (2017) 17:215 DOI 10.1186/s12862-017-1061-5 RESEARCH ARTICLE Open Access Conservation and diversification of small RNA pathways within flatworms Santiago Fontenla1, Gabriel Rinaldi2, Pablo Smircich1,3 and Jose F. Tort1* Abstract Background: Small non-coding RNAs, including miRNAs, and gene silencing mediated by RNA interference have been described in free-living and parasitic lineages of flatworms, but only few key factors of the small RNA pathways have been exhaustively investigated in a limited number of species. The availability of flatworm draft genomes and predicted proteomes allowed us to perform an extended survey of the genes involved in small non-coding RNA pathways in this phylum. Results: Overall, findings show that the small non-coding RNA pathways are conserved in all the analyzed flatworm linages; however notable peculiarities were identified. While Piwi genes are amplified in free-living worms they are completely absent in all parasitic species. Remarkably all flatworms share a specific Argonaute family (FL-Ago) that has been independently amplified in different lineages. Other key factors such as Dicer are also duplicated, with Dicer-2 showing structural differences between trematodes, cestodes and free-living flatworms. Similarly, a very divergent GW182 Argonaute interacting protein was identified in all flatworm linages. Contrasting to this, genes involved in the amplification of the RNAi interfering signal were detected only in the ancestral free living species Macrostomum lignano. We here described all the putative small RNA pathways present in both free living and parasitic flatworm lineages. Conclusion: These findings highlight innovations specifically evolved in platyhelminths presumably associated with novel mechanisms of gene expression regulation mediated by small RNA pathways that differ to what has been classically described in model organisms. -
Agent for Expelling Parasites in Humans, Animals Or Birds
(19) TZZ Z_T (11) EP 2 496 089 B1 (12) EUROPEAN PATENT SPECIFICATION (45) Date of publication and mention (51) Int Cl.: of the grant of the patent: A01N 65/00 (2009.01) A01N 65/10 (2009.01) 22.02.2017 Bulletin 2017/08 A61K 36/23 (2006.01) A01P 5/00 (2006.01) (21) Application number: 10803029.7 (86) International application number: PCT/BE2010/000077 (22) Date of filing: 05.11.2010 (87) International publication number: WO 2011/054066 (12.05.2011 Gazette 2011/19) (54) AGENT FOR EXPELLING PARASITES IN HUMANS, ANIMALS OR BIRDS MITTEL ZUR ABWEISUNG VON PARASITEN BEI MENSCHEN, TIEREN ODER VÖGELN AGENT POUR EXPULSER DES PARASITES CHEZ DES HUMAINS, DES ANIMAUX OU DES OISEAUX (84) Designated Contracting States: (56) References cited: AL AT BE BG CH CY CZ DE DK EE ES FI FR GB • RAMADAN NASHWA I ET AL: "The in vitro effect GR HR HU IE IS IT LI LT LU LV MC MK MT NL NO of assafoetida on Trichomonas vaginalis", PL PT RO RS SE SI SK SM TR JOURNAL OF THE EGYPTIAN SOCIETY OF PARASITOLOGY, EGYPTIAN SOCIETY OF (30) Priority: 06.11.2009 BE 200900689 PARAS1TOLOGY, CAIRO, EG, vol. 33, no. 2, 1 August 2003 (2003-08-01) , pages 615-630, (43) Date of publication of application: XP009136264, ISSN: 1110-0583 12.09.2012 Bulletin 2012/37 • DATABASE MEDLINE [Online] US NATIONAL LIBRARY OF MEDICINE (NLM), BETHESDA, MD, (73) Proprietors: US; December 2004 (2004-12), RAMADAN • MEIJS, Maria Wilhelmina NASHWA I ET AL: "Effect of Ferula assafoetida 4852 Hombourg (BE) on experimental murine Schistosoma mansoni • VAESSEN, Jan Jozef infection.", XP002592455, Database accession 4852 Hombourg (BE) no. -
Fibre Couplings in the Placenta of Sperm Whales, Grows to A
news and views Most (but not all) nematodes are small Daedalus and nondescript. For example, Placento- T STUDIOS nema gigantissima, which lives as a parasite Fibre couplings in the placenta of sperm whales, grows to a CS./HOL length of 8 m, with a diameter of 2.5 cm. The The nail, says Daedalus, is a brilliant and free-living, marine Draconema has elongate versatile fastener, but with a fundamental O ASSO T adhesive organs on the head and along the contradiction. While being hammered in, HO tail, and moves like a caterpillar. But the gen- it is a strut, loaded in compression. It must BIOP eral uniformity of most nematode species be thick enough to resist buckling. Yet has hampered the establishment of a classifi- once in place it is a tie, loaded in tension, 8 cation that includes both free-living and par- and should be thin and flexible to bear its asitic species. Two classes have been recog- load efficiently. He is now resolving this nized (the Secernentea and Adenophorea), contradiction. based on the presence or absence of a caudal An ideal nail, he says, should be driven sense organ, respectively. But Blaxter et al.1 Figure 2 The bad — eelworm (root knot in by a force applied, not to its head, but to have concluded from the DNA sequences nematode), which forms characteristic nodules its point. Its shaft would then be drawn in that the Secernentea is a natural group within on the roots of sugar beet and rice. under tension; it could not buckle, and the Adenophorea. -
Hymenolepis Nana Is a Ubiquitous Parasite, Found Throughout Many Developing and Developed Countries
Characterisation of Community-Derived Hymenolepis Infections in Australia Marion G. Macnish BSc. (Medical Science) Hons Division of Veterinary and Biomedical Sciences Murdoch University Western Australia This thesis is presented for the degree of Doctor of Philosophy of Murdoch University 2001 I declare that this thesis is my own account of my research and contains as its main work which has not been submitted for a degree at any other educational institution. ………………………………………………. (Marion G. Macnish) Characterisation of Community-Derived Hymenolepis Infections in Australia ii Abstract Hymenolepis nana is a ubiquitous parasite, found throughout many developing and developed countries. Globally, the prevalence of H. nana is alarmingly high, with estimates of up to 75 million people infected. In Australia, the rates of infection have increased substantially in the last decade, from less than 20% in the early 1990’s to 55 - 60% in these same communities today. Our knowledge of the epidemiology of infection of H. nana is hampered by the confusion surrounding the host specificity and taxonomy of this parasite. The suggestion of the existence of two separate species, Hymenolepis nana von Siebold 1852 and Hymenolepis fraterna Stiles 1906, was first proposed at the beginning of the 20th century. Despite ongoing discussions in the subsequent years it remained unclear, some 90 years later, whether there were two distinct species, that are highly host specific, or whether they were simply the same species present in both rodent and human hosts. The ongoing controversy surrounding the taxonomy of H. nana has not yet been resolved and remains a point of difference between the taxonomic and medical literature. -
Epidemiology of Angiostrongylus Cantonensis and Eosinophilic Meningitis
Epidemiology of Angiostrongylus cantonensis and eosinophilic meningitis in the People’s Republic of China INAUGURALDISSERTATION zur Erlangung der Würde eines Doktors der Philosophie vorgelegt der Philosophisch-Naturwissenschaftlichen Fakultät der Universität Basel von Shan Lv aus Xinyang, der Volksrepublik China Basel, 2011 Genehmigt von der Philosophisch-Naturwissenschaftlichen Fakult¨at auf Antrag von Prof. Dr. Jürg Utzinger, Prof. Dr. Peter Deplazes, Prof. Dr. Xiao-Nong Zhou, und Dr. Peter Steinmann Basel, den 21. Juni 2011 Prof. Dr. Martin Spiess Dekan der Philosophisch- Naturwissenschaftlichen Fakultät To my family Table of contents Table of contents Acknowledgements 1 Summary 5 Zusammenfassung 9 Figure index 13 Table index 15 1. Introduction 17 1.1. Life cycle of Angiostrongylus cantonensis 17 1.2. Angiostrongyliasis and eosinophilic meningitis 19 1.2.1. Clinical manifestation 19 1.2.2. Diagnosis 20 1.2.3. Treatment and clinical management 22 1.3. Global distribution and epidemiology 22 1.3.1. The origin 22 1.3.2. Global spread with emphasis on human activities 23 1.3.3. The epidemiology of angiostrongyliasis 26 1.4. Epidemiology of angiostrongyliasis in P.R. China 28 1.4.1. Emerging angiostrongyliasis with particular consideration to outbreaks and exotic snail species 28 1.4.2. Known endemic areas and host species 29 1.4.3. Risk factors associated with culture and socioeconomics 33 1.4.4. Research and control priorities 35 1.5. References 37 2. Goal and objectives 47 2.1. Goal 47 2.2. Objectives 47 I Table of contents 3. Human angiostrongyliasis outbreak in Dali, China 49 3.1. Abstract 50 3.2. -
SR-109: Strongyles in Horses
AGRICULTURAL EXPERIMENT STATION UNIVERSITY OF KENTUCKY COLLEGE OF AGRICULTURE, FOOD AND ENVIRONMENT, LEXINGTON, KY, 40546 SR-109 Strongyles in Horses Update 2015 E.T. Lyons and S.C. Tolliver, Veterinary Science Introduction Parasites live in a host from which they obtain food and pro- tection. They may harm but usu- ally do not benefit the host. The Mature worms word “parasite” is derived from the Immature worms live in Latin and Greek languages mean- migrate in tissues intestinal tract ing, in general, “one who eats at the table of another.” It is said that Stages inside the horse a “good” parasite does not overtly harm or kill its host. It is theoreti- Stages outside the horse cally possible that a more benign parasite (e.g. Gasterophilus spp.) is Infective stages much “older in eons of time” and ingested in food and water Eggs passed it and its host have adjusted better in feces to each other than a conceivably “newer” parasite (e.g. Strongylus spp.) which may be more harmful Infective larvae to its host. develop Taxonomy Horses can harbor over 100 species of internal parasites. About Figure 1. Strongyle life cycle. one half of these species are nema- todes in the strongyle group (fam- ily Strongylidae Baird, 1853). They the genera Bidentostomum Tshoijo to the first stage larva (L1) which are separated taxonomically into in Popova, 1958, Craterostomum hatches and then develops to the two categories—large strongyles Boulenger, 1920, Oesophagodon- second stage larva (L2), and finally (subfamily Strongylinae Railliet, tus Railliet et Henry, 1902, and to the third stage larva (L3) which 1893) and small strongyles (cya- Triodontophorus Looss, 1902. -
Editorial Be Careful What You Eat!
Am. J. Trop. Med. Hyg., 101(5), 2019, pp. 955–956 doi:10.4269/ajtmh.19-0595 Copyright © 2019 by The American Society of Tropical Medicine and Hygiene Editorial Be Careful What You Eat! Philip J. Rosenthal* Department of Medicine, University of California, San Francisco, California The readership of the American Journal of Tropical ingestion of live centipedes.4 Centipedes purchased from the Medicine and Hygiene is well acquainted with the risks of same market used by the patients contained A. cantonensis infectious diseases acquired from foods contaminated with larvae; thus, in addition to slugs, snails, and some other pathogenic viruses, bacteria, protozoans, or helminths due to studied invertebrates, centipedes may be an intermediate improper hygiene. Less familiar may be uncommon infections host for the parasite. The patients appeared to respond to associated with ingestion of unusual uncooked foods, eaten ei- treatment with albendazole and dexamethasone. The value of ther purposely or inadvertantly. A number of instructive examples treatment, which might exacerbate meningitis due to dying have been published in the Journal within the last 2 years; these worms, has been considered uncertain; a recent perspective all involve helminths for which humans are generally not the de- also published in the Journal suggested that treatment early finitive host, but can become ill when they unwittingly become after presentation with disease is advisable to prevent pro- accidental hosts after ingestion of undercooked animal products. gression of illness, including migration of worms to the lungs.5 This issue of the Journal includes two reports on cases of Ingestion of raw centipedes is best avoided. -
Molecular Characterization of Β-Tubulin Isotype-1 Gene of Bunostomum Trigonocephalum
Int.J.Curr.Microbiol.App.Sci (2018) 7(7): 3351-3358 International Journal of Current Microbiology and Applied Sciences ISSN: 2319-7706 Volume 7 Number 07 (2018) Journal homepage: http://www.ijcmas.com Original Research Article https://doi.org/10.20546/ijcmas.2018.707.390 Molecular Characterization of β-Tubulin Isotype-1 Gene of Bunostomum trigonocephalum Ravi Kumar Khare1, A. Dixit3, G. Das4, A. Kumar1, K. Rinesh3, D.S. Khare4, D. Bhinsara1, Mohar Singh2, B.C. Parthasarathi2, P. Dipali2, M. Shakya5, J. Jayraw5, D. Chandra2 and M. Sankar1* 1Division of Temperate Animal Husbandry, ICAR- IVRI, Mukteswar, India 2IVRI, Izatnagar, India 3College of Veterinary Science and A.H., Rewa, India 4College of Veterinary Sciences and A.H., Jabalpur, India 5College of Veterinary Sciences and A.H., Mhow, India *Corresponding author ABSTRACT The mechanism of benzimidazoles resistance is linked to single nucleotide polymorphisms (SNPs) on beta -tubulin isotype-1 gene. The three known SNPs responsible for BZ K e yw or ds resistance are F200Y, F167Y and E198A on the beta-tubulin isotype-1. The present study was aimed to characterize beta-tubulin isotype-1 gene of Bunostomum trigonocephalum, Benzimidazole for identifying variations on possible mutation sites. The adult parasites were collected resistance, Beta from Mukteswar, Uttarakhand. The parasites were thoroughly examined morphologically tubulin, and male parasites were subjected for RNA isolation. Complementary DNA (cDNA) was Bunostomum synthesised from total RNA using OdT. The PCR was performed using cDNA and self trigonocephalum, Small ruminants designed degenerative primers. The purified PCR amplicons were cloned into pGEMT easy vector and custom sequenced. The obtained sequences were analysed using DNA Article Info STAR, MEGA7.0 and Gene tool software. -
The Mitochondrial Genome of the Soybean Cyst Nematode, Heterodera Glycines
565 The mitochondrial genome of the soybean cyst nematode, Heterodera glycines Tracey Gibson, Daniel Farrugia, Jeff Barrett, David J. Chitwood, Janet Rowe, Sergei Subbotin, and Mark Dowton Abstract: We sequenced the entire coding region of the mitochondrial genome of Heterodera glycines. The sequence ob- tained comprised 14.9 kb, with PCR evidence indicating that the entire genome comprised a single, circular molecule of ap- proximately 21–22 kb. The genome is the most T-rich nematode mitochondrial genome reported to date, with T representing over half of all nucleotides on the coding strand. The genome also contains the highest number of poly(T) tracts so far reported (to our knowledge), with 60 poly(T) tracts ≥ 12 Ts. All genes are transcribed from the same mitochon- drial strand. The organization of the mitochondrial genome of H. glycines shows a number of similarities compared with Ra- dopholus similis, but fewer similarities when compared with Meloidogyne javanica. Very few gene boundaries are shared with Globodera pallida or Globodera rostochiensis. Partial mitochondrial genome sequences were also obtained for Hetero- dera cardiolata (5.3 kb) and Punctodera chalcoensis (6.8 kb), and these had identical organizations compared with H. gly- cines. We found PCR evidence of a minicircular mitochondrial genome in P. chalcoensis, but at low levels and lacking a noncoding region. Such circularised genome fragments may be present at low levels in a range of nematodes, with multipar- tite mitochondrial genomes representing a shift to a condition in which these subgenomic circles predominate. Key words: mitochondrial, nematode, gene rearrangement, Punctodera, Punctoderinae, Heteroderidae, Heterodera cardio- lata.