Gas-Phase Reaction of Trans-2-Methyl-2-Butenal with Cl: Kinetics, Gaseous Products, and SOA Formation
Total Page:16
File Type:pdf, Size:1020Kb
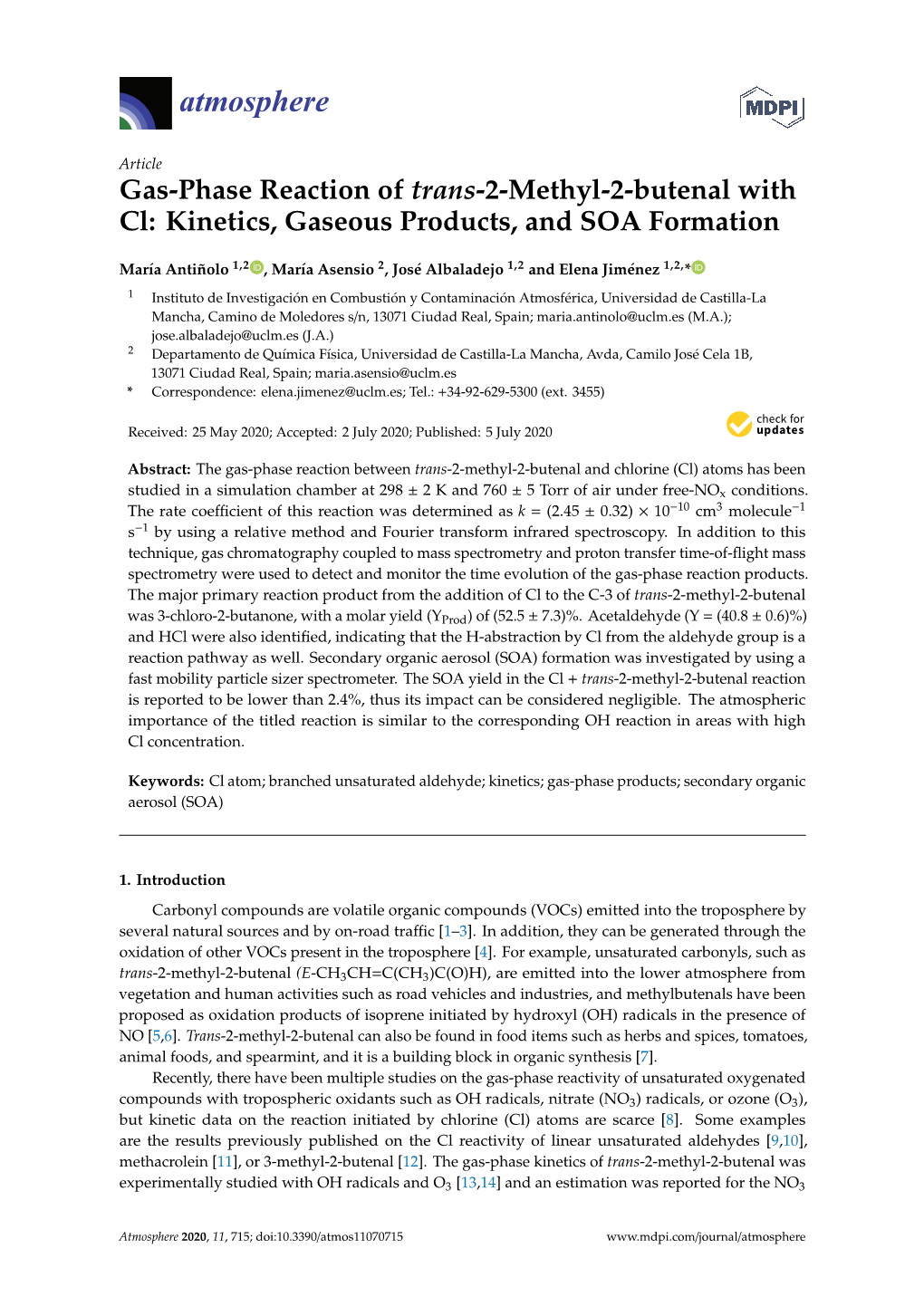
Load more
Recommended publications
-
Guidelines to Achieving High Selectivity for the Hydrogenation of Α
Guidelines to achieving high selectivity for the hydrogenation of α,β-unsaturated aldehydes with bimetallic and dilute alloy catalysts − A review Mathilde Luneau,†,a Jin Soo Lim,†,a Dipna A. Patel,‡,a E. Charles H. Sykes,‡ Cynthia M. Friend,† and Philippe Sautet*,,§ † Department of Chemistry and Chemical Biology, Harvard University, Cambridge, MA 02138, USA ‡ Department of Chemistry, Tufts University, Medford, MA 02155, USA Department of Chemical and Biomolecular Engineering, University of California, Los Angeles, Los Angeles, CA 90095, USA § Department of Chemistry and Biochemistry, University of California, Los Angeles, Los Angeles, CA 90095, USA * Email: [email protected] a These three authors contributed equally to this review. Abstract Selective hydrogenation of α,ß-unsaturated aldehydes to unsaturated alcohols is a challenging class of reactions, yielding valuable intermediates for the production of pharmaceuticals, perfumes, and flavorings. On monometallic heterogeneous catalysts, the formation of the unsaturated alcohols is thermodynamically disfavored over the saturated aldehydes. Hence, new catalysts are required to achieve the desired selectivity. Herein, the literature of three major research areas in catalysis is integrated as a step toward establishing the guidelines for enhancing the selectivity: reactor studies of complex catalyst materials at operating temperature and pressure; surface science studies of crystalline surfaces in ultrahigh vacuum; and first-principles modeling using density functional theory calculations. Aggregate analysis shows that bimetallic and dilute alloy catalysts significantly enhance the selectivity to the unsaturated alcohols compared to monometallic catalysts. This com- prehensive review focuses primarily on the role of different metal surfaces as well as the factors that promote the adsorption of the unsaturated aldehyde via its C=O bond, most notably by elec- tronic modification of the surface and formation of the electrophilic sites. -
Qt4p41x6ph.Pdf
UC Irvine UC Irvine Previously Published Works Title Rate constants for the reactions of chlorine atoms with a series of unsaturated aldehydes and ketones at 298 K: structure and reactivity Permalink https://escholarship.org/uc/item/4p41x6ph Journal Physical Chemistry Chemical Physics, 4(10) ISSN 14639076 14639084 Authors Wang, Weihong Ezell, Michael J Ezell, Alisa A et al. Publication Date 2002-05-01 DOI 10.1039/b111557j Peer reviewed eScholarship.org Powered by the California Digital Library University of California View Article Online / Journal Homepage / Table of Contents for this issue PCCP Rate constants for the reactions of chlorine atoms with a series of unsaturated aldehydes and ketones at 298 K: structure and reactivity Weihong Wang, Michael J. Ezell, Alisa A. Ezell, Gennady Soskin and Barbara J. Finlayson-Pitts* Department of Chemistry, University of California, Irvine, CA 92697-2025. E-mail: bjfi[email protected]; Fax: 949 824-3168; Tel: 949 824-7670 Received 2nd January 2002, Accepted 31st January 2002 First published as an Advance Article on the web 18th April 2002 The kinetics and mechanisms of chlorine atom reactions with the products of organic oxidations in the atmosphere are of interest for understanding the chemistry of coastal areas. We report here the first kinetics measurements of the reactions of atomic chlorine with 4-chlorocrotonaldehyde and chloromethyl vinyl ketone, recently identified as products of the reaction of chlorine atoms with 1,3-butadiene. The reactions with acrolein, methacrolein, crotonaldehyde, methyl vinyl ketone and crotyl chloride were also studied to probe structure- reactivity relationships. Relative rate studies were carried out at 1 atm and 298 K using two different approaches: long path FTIR for the acrolein, methacrolein, crotonaldehyde and methyl vinyl ketone reactions with acetylene as the reference compound, and a collapsible Teflon reaction chamber with GC-FID detection of the organics using n-butane or n-nonane as the reference compounds for the entire series. -
Alkene Metathesis for Transformations of Renewables Christian Bruneau, Cedric Fischmeister
Alkene Metathesis for Transformations of Renewables Christian Bruneau, Cedric Fischmeister To cite this version: Christian Bruneau, Cedric Fischmeister. Alkene Metathesis for Transformations of Renewables. Organometallics for Green Catalysis, Springer, pp.77-102, 2018, 10.1007/3418_2018_18. hal- 01940683 HAL Id: hal-01940683 https://hal-univ-rennes1.archives-ouvertes.fr/hal-01940683 Submitted on 30 Nov 2018 HAL is a multi-disciplinary open access L’archive ouverte pluridisciplinaire HAL, est archive for the deposit and dissemination of sci- destinée au dépôt et à la diffusion de documents entific research documents, whether they are pub- scientifiques de niveau recherche, publiés ou non, lished or not. The documents may come from émanant des établissements d’enseignement et de teaching and research institutions in France or recherche français ou étrangers, des laboratoires abroad, or from public or private research centers. publics ou privés. Alkene Metathesis for Transformations of Renewables Christian Bruneau and Cédric Fischmeister 1 Introduction With the depletion of fossil resources and the concerns about climate chang- ing and environment protection, biomass is intensively considered as a sus- tainable source of raw material for the chemical industry and for the produc- tion of biofuels.[1-4] Beside the very abundant carbohydrate and lignocellulosic biomass, lipids and to a lesser extend terpenes are envisioned as promising candidates for the production of bio-sourced compounds with a broad range of applications.[5,6] Terpenes are of most importance in fra- grance composition whereas fats and oils have already found application as bio-diesel fuel and they are also foreseen as a renewable source of polymers. -
Efficient Synthesis of Methyl Methacrylate by One Step Oxidative
catalysts Article Efficient Synthesis of Methyl Methacrylate by One Step Oxidative Esterification over Zn-Al-Mixed Oxides Supported Gold Nanocatalysts Huayin Li 1, Yuan Tan 1,* , Xingkun Chen 1, Wenshao Yang 1, Chuanqi Huang 1, Jie Li 1 and Yunjie Ding 1,2,3,* 1 Hangzhou Institute of Advanced studies, Zhejiang Normal University, Hangzhou 311231, China; [email protected] (H.L.); [email protected] (X.C.); [email protected] (W.Y.); [email protected] (C.H.); [email protected] (J.L.) 2 Dalian National Laboratory for Clean Energy, Dalian Institute of Chemical Physics, Chinese Academy of Sciences, Dalian 116023, China 3 The State Key Laboratory of Catalysis, Dalian Institute of Chemical Physics, Chinese Academy of Sciences, Dalian 116023, China * Correspondence: [email protected] (Y.T.); [email protected] (Y.D.); Tel.: +86-571-82257902 (Y.T.); +86-411-84379143 (Y.D.) Abstract: Methyl methacrylate (MMA) is an important monomer in fine chemicals. The synthesis of MMA by one-step oxidative esterification from methacrolein with methanol over a heterogeneous catalyst with high activity, selectivity and stability is highly desirable. Herein, Zn-Al-hydrotalcites 2+ 3+ (HTs)-supported atomically precise Au25 nanoclusters with different molar ratios of Zn /Al were prepared and used as the precursors for this reaction. They exhibited good performances in com- parison with the gold catalysts prepared by the deposition precipitation method. The structural and electronic properties were evaluated by various characterization technologies, including X-ray diffraction (XRD), high-resolution transmission electron microscopy (HRTEM), in situ diffuse re- Citation: Li, H.; Tan, Y.; Chen, X.; Yang, W.; Huang, C.; Li, J.; Ding, Y. -
Aqueous Phase Photooxidation
Atmos. Chem. Phys., 9, 5093–5105, 2009 www.atmos-chem-phys.net/9/5093/2009/ Atmospheric © Author(s) 2009. This work is distributed under Chemistry the Creative Commons Attribution 3.0 License. and Physics In-cloud processes of methacrolein under simulated conditions – Part 1: Aqueous phase photooxidation Yao Liu1, I. El Haddad1, M. Scarfogliero2, L. Nieto-Gligorovski1, B. Temime-Roussel1, E. Quivet1, N. Marchand1, B. Picquet-Varrault2, and A. Monod1 1Universites´ d’Aix-Marseille I, II et III – CNRS, UMR 6264, Laboratoire Chimie Provence, Equipe: IRA, 3 place Victor Hugo, 13331 Marseilles Cedex 3, France 2Laboratoire Interuniversitaire des Systemes` Atmospheriques´ (UMR 7583), Universite´ Paris, France Received: 22 January 2009 – Published in Atmos. Chem. Phys. Discuss.: 10 March 2009 Revised: 30 June 2009 – Accepted: 1 July 2009 – Published: 28 July 2009 Abstract. The photooxidation of methacrolein was stud- phase (Monod et al., 2005). These compounds readily par- ied in the aqueous phase under simulated cloud droplet tition into the droplets, and oxidize further in the aqueous conditions. The obtained rate constant of OH-oxidation phase to form less volatile organics. Several experimen- of methacrolein at 6◦C in unbuffered solutions was tal and modelling studies have demonstrated that aldehydes 5.8(±0.9)×109 M−1 s−1. The measured rate coefficient is such as glyoxal, methylglyoxal and glycolaldehyde can form consistent with OH-addition on the C=C bond. This was low volatility products such as glyoxylic and oxalic acids as confirmed by the mechanism established on the study of the well as larger molecular weight compounds and oligomers by reaction products (at 25◦C in unbuffered solutions) where aqueous phase reactions (Warneck, 2003; Altieri et al., 2006, methylglyoxal, formaldehyde, hydroxyacetone and acetic 2008; Carlton et al., 2007). -
The Selective Hydrogenation of Crotonaldehyde Over Bimetallic Catalysts Ann Marie Schoeb Iowa State University
Iowa State University Capstones, Theses and Retrospective Theses and Dissertations Dissertations 1996 The selective hydrogenation of crotonaldehyde over bimetallic catalysts Ann Marie Schoeb Iowa State University Follow this and additional works at: https://lib.dr.iastate.edu/rtd Part of the Chemical Engineering Commons Recommended Citation Schoeb, Ann Marie, "The es lective hydrogenation of crotonaldehyde over bimetallic catalysts " (1996). Retrospective Theses and Dissertations. 11413. https://lib.dr.iastate.edu/rtd/11413 This Dissertation is brought to you for free and open access by the Iowa State University Capstones, Theses and Dissertations at Iowa State University Digital Repository. It has been accepted for inclusion in Retrospective Theses and Dissertations by an authorized administrator of Iowa State University Digital Repository. For more information, please contact [email protected]. INFORMATION TO USERS This manuscript has been reproduced from the microfihn master. UMI films the text directly from the original or copy submitted. Thus, some thesis and dissertation copies are in typewriter &ce, while others may be from any type of computer printer. The quality of this reproduction is dependent upon the quality of the copy submitted. Broken or indistinct print, colored or poor quality illustrations and photographs, print bleedthrough, substandard margins, and improper alignment can adversely affect reproduction. In the unlikely event that the author did not send UMI a complete manuscript and there are missing pages, these will be noted. Also, if unauthorized copyright material had to be removed, a note will indicate the deletion. Oversize materials (e.g., maps, drawings, charts) are reproduced by sectioning the original, beginning at the upper left-hand comer and continuing from left to right in equal sections with small overlaps. -
Selective Oxidation of Isobutane to Methacrylic Acid and Methacrolein: a Critical Review
catalysts Review Selective Oxidation of Isobutane to Methacrylic Acid and Methacrolein: A Critical Review Li Zhang ,Sébastien Paul , Franck Dumeignil * and Benjamin Katryniok * University Lille, CNRS, Centrale Lille, University Artois, UMR 8181–UCCS–Unité de Catalyse et Chimie du Solide, F-59000 Lille, France; [email protected] (L.Z.); [email protected] (S.P.) * Correspondence: [email protected] (F.D.); [email protected] (B.K.) Abstract: Selective oxidation of isobutane to methacrolein (MAC) and methacrylic acid (MAA) has received great interest both in the chemical industry and in academic research. The advantages of this reaction originate not only from the low cost of the starting material and reduced process complexity, but also from limiting the use of toxic reactants and the production of wastes. Successive studies and reports have shown that heteropolycompounds (HPCs) with Keggin structure (under the form of partially neutralized acids with increased stability) can selectively convert isobutane to MAA and MAC due to their strong and tunable acidity and redox properties. This review hence aims to discuss the Keggin-type HPCs that have been used in recent years to catalyze the oxidation of isobutane to MAA and MAC, and to review alternative metal oxides with proper redox properties for the same reaction. In addition, the influence of the main reaction conditions will be discussed. Keywords: Keggin-type HPCs; isobutane; oxidation; methacrolein; methacrylic acid Citation: Zhang, L.; Paul, S.; 1. Introduction Dumeignil, F.; Katryniok, B. Selective Hydrocarbons are generally issued from coal, oil, and natural gas. They are still the Oxidation of Isobutane to Methacrylic main feedstock of the chemical industry. -
Mechanism of the Hydroxyl Radical Oxidation of Methacryloyl Peroxynitrate (Mpan) and Its Pathway Toward Secondary Organic Aerosol Formation in the Atmosphere
335 Appendix C MECHANISM OF THE HYDROXYL RADICAL OXIDATION OF METHACRYLOYL PEROXYNITRATE (MPAN) AND ITS PATHWAY TOWARD SECONDARY ORGANIC AEROSOL FORMATION IN THE ATMOSPHERE Nguyen, T. B., K. H. Bates, J. D. Crounse, R. H. Schwantes, X. Zhang, H. G. Kjaergaard, J. D. Surratt, P. Lin, A. Laskin, J. H. Seinfeld, and P. O. Wennberg (2015). “Mechanism of the hydroxyl radical oxidation of methacryloyl perox- ynitrate (MPAN) and its pathway toward secondary organic aerosol formation in the atmosphere”. In: Phys. Chem. Chem. Phys. 17.27, pp. 17914–17926. : 10.1039/C5CP02001H. Abstract Methacryloyl peroxynitrate (MPAN), the acyl peroxynitrate of methacrolein, has been suggested to be an important secondary organic aerosol (SOA) precursor from isoprene oxidation, but the mechanism by which MPAN produces SOA through re- action with the hydroxyl radical (OH) remains unclear. We systematically evaluate three proposed mechanisms in controlled chamber experiments and provide the first experimental support for the theoretically-predicted lactone formation pathway from the MPAN + OH reaction, producing hydroxymethyl-methyl-↵-lactone (HMML). The decomposition of the MPAN-OH adduct yields HMML + NO ( 75%) and 3 ⇠ hydroxyacetone + CO + NO ( 25%), out-competing its reaction with atmospheric 3 ⇠ oxygen. The production of other proposed SOA precursors, e.g. methacrylic acid epoxide (MAE), from MPAN and methacrolein are negligible (<2%). Furthermore, we show that the β-alkenyl moiety of MPAN is critical for lactone formation. Alkyl radicals formed cold via H-abstraction by OH do not decompose to HMML, even if they are structurally identical to the MPAN-OH adduct. The SOA formation from HMML, from polyaddition of the lactone to organic compounds at the particle in- terface or in the condensed phase, is close to unity under dry conditions. -
Supplementary Information
Supplementary Information Figure S1. Soil moisture and total OH reactivity in hourly time resolution for the four measurement periods. 5 Figure S2. Unattributed OH reactivity fraction profiles for noon- and nighttime in each measurement period. The shaded areas represent the standard deviation. The measurement uncertainty given is the average over all observation periods. Note that the unattributed fraction is usually below the measurement uncertainty. 1 Figure S3. Campaign average noontime total OH reactivities as a function of height with linear regressions for gradient determination. The results of the linear regressions were: March 2018: R = -0.005*h + 25.7; October 2018: R = -0.015*h + 34.0; 5 June 2019: R = -0.016*h + 24.3; September 2019: R = -0.026*h + 40.4. 10 Figure S4. Modelled total OH reactivity from the temperature-dependent parameterization (Sect. 3.3.1) vs. measured total OH reactivity. Linear regression: y = 8.7 + 0.6x, r² = 0.61. 2 Figure S5. Timeseries of meteorological parameters (wind speed at 320 m, precipitation at 320 m, PAR at 80 m), OVOC OH reactivity and acetaldehyde mixing ratios around (a) a daytime rain event and (b) an evening rain event. (c) Vertical profiles of 5 OVOC-attributed OH reactivity for two rain events. 3 0.2 0.4 0.6 0.8 -3 40 Black carbon (µg m ) ) -1 30 20 Total OH reactivity (s 10 5 10 15 20 25 -1 Isoprene OH reactivity (s ) Figure S6. Total OH reactivity as a function of terpenoid reactivity with black carbon mass concentration as a colour scale for September 2019. -
Methacrylic Acid and Methacrylic Esters
PROCESS ECONOMICS PROGRAM ..-. SRI INTERNATIONAL Mm10 Park, California Abstract 9402E Process Economics Program Report No. 11C METHACRYLIC ACID AND METHACRYLIC ESTERS (September 1984) For the last fifteen years, processes have been developed to supplant the established process for making methyl methacrylate from acetone, hydrogen cyanide, and methanol. The acetone cyanohydrin route suffers from production of acidic wastes; the newer processes avoid these wastes and offer lower costs. Since our most recent previous report on the subject (Report LlB, issued in July 1980), a methyl methacrylate process based on a C4 feed- stock has been commercialized in Japan. This process oxidizes a t-butanol feed to methacrolein and methacrylic acid in two stages, then esterifies the acid to methyl methacrylate. We have evaluated this process and several variations, including the alternative use of isobutane feed and the supplementary use of isobutyraldehyde (by-product from 0x0 alcohol plants). We have also evaluated an attractive process based on propylene hydrocarboxylation to isobutyric acid, followed by dehydrogenation to methacrylic acid. We have updated some of the processes evaluated in Report llB, including the acetone cyanohydrin process. For a newly constructed plant, and at market prices for HCN and acetone, the acetone cyanohydrin process is more expensive than several of the newer processes. PEP'83 RHS - ReportNo. 11C a METHACRYLIC ACID AND ESTERS SUPPLEMENT C by ROBERT H. SCHWAAR ETSUO MATSUNAGA SHOICHI KIKUCHI a- and WATARU NISHIMOTO September 1984 - Cl a A private report by the PROCESS ECONOMICS PROGRAM a m Menlo Park, California 94025 For detailed marketing data and information, the reader is referred to one of the SRI programs specializing in marketing research. -
Role of Aldehyde Chemistry and NO Concentrations in Secondary
Atmos. Chem. Phys. Discuss., 10, 10219–10269, 2010 Atmospheric www.atmos-chem-phys-discuss.net/10/10219/2010/ Chemistry © Author(s) 2010. This work is distributed under and Physics the Creative Commons Attribution 3.0 License. Discussions This discussion paper is/has been under review for the journal Atmospheric Chemistry and Physics (ACP). Please refer to the corresponding final paper in ACP if available. Role of aldehyde chemistry and NOx concentrations in secondary organic aerosol formation A. W. H. Chan1, M. N. Chan2, J. D. Surratt1, P. S. Chhabra1, C. L. Loza1, J. D. Crounse1, L. D. Yee2, R. C. Flagan1,2, P. O. Wennberg2,3, and J. H. Seinfeld1,2 1Division of Chemistry and Chemical Engineering, California Institute of Technology, Pasadena, CA, USA 2Division of Engineering and Applied Science, California Institute of Technology, Pasadena, CA, USA 3Division of Geological and Planetary Sciences, California Institute of Technology, Pasadena, CA, USA Received: 7 April 2010 – Accepted: 12 April 2010 – Published: 19 April 2010 Correspondence to: J. H. Seinfeld ([email protected]) Published by Copernicus Publications on behalf of the European Geosciences Union. 10219 Abstract Aldehydes are an important class of products from atmospheric oxidation of hy- drocarbons. Isoprene (2-methyl-1,3-butadiene), the most abundantly emitted atmo- spheric non-methane hydrocarbon, produces a significant amount of secondary or- 5 ganic aerosol (SOA) via methacrolein (a C4-unsaturated aldehyde) under urban high- NOx conditions. Previously, we have identified peroxy methacryloyl nitrate (MPAN) as the important intermediate to isoprene and methacrolein SOA in this NOx regime. Here we show that as a result of this chemistry, NO2 enhances SOA formation from methacrolein and two other α,β-unsaturated aldehydes, specifically acrolein and cro- 10 tonaldehyde, a NOx effect on SOA formation previously unrecognized. -
Stereoselectivity in Atmospheric Autoxidation
Supporting Information for: Stereoselectivity in Atmospheric Autoxidation Kristian H. Møller,y Eric Praske,z Lu Xu,{ John D. Crounse,{ Paul O. Wennberg,*,{,x and Henrik G. Kjaergaard*,y yDepartment of Chemistry, University of Copenhagen, DK-2100 Copenhagen Ø, Denmark zDivision of Chemistry and Chemical Engineering, California Institute of Technology, Pasadena, California 91125, United States {Division of Geological and Planetary Sciences, California Institute of Technology, Pasadena, California 91125, United States xDivision of Engineering and Applied Science, California Institute of Technology, Pasadena, California 91125, United States *To whom correspondence should be addressed. Contents S1 Theoretical Methods S4 S2 Assessment of Conformational Sampling S5 S3 Calculated H-shift Rate Contants and Associated Data S6 S3.1 2-Methylpropene . S6 S3.2 Crotonaldehyde (CRALD) . S8 S3.3 Acrolein (ACR) . S12 S3.4 Methacrolein (MACR) . S14 S4 Comparison of Aldehydic H-shifts in Crotonaldehyde, Methacrolein and Acrolein S16 S5 Temperature Dependence of Calculated Aldehydic H-shift Rate Coefficients S17 S6 Alkyl Radical Stability S17 S7 Comparison of Alkoxy Bond Scissions S18 S8 Determination of CIMS Sensitivities S18 S9 Experimental Methods S20 S10 Initial Mixing Ratios S23 S11 Determination of Bimolecular Lifetime S23 S12 Importance of RO2 + RO2 S25 S13 Simple Chemical Model to Obtain Experimental H-shift Rate Coefficients S26 S14 Uncertainty in the Experimental Reaction Rate Coefficients S28 S14.1 Uncertainty in Peak Area Ratio . S28 S14.2 Uncertainty