Adopting Multifunctional Material Systems
Total Page:16
File Type:pdf, Size:1020Kb
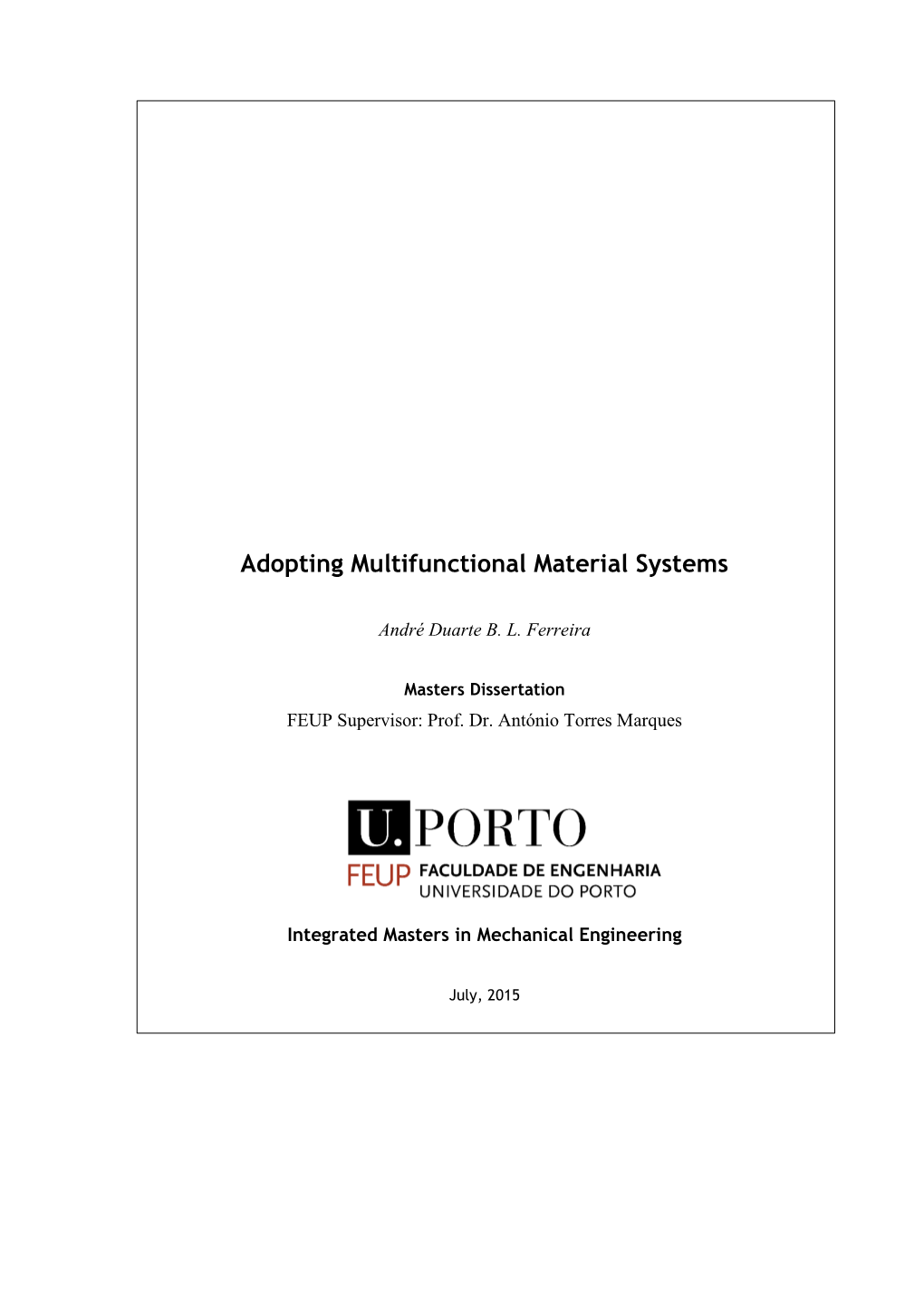
Load more
Recommended publications
-
Analýza Nejposlouchanějších Skladeb Na Serveru Youtube.Com
Masarykova univerzita Pedagogická fakulta ANALÝZA NEJPOSLOUCHANĚJŠÍCH SKLADEB NA SERVERU YOUTUBE.COM Bakalářská práce Brno 2017 Autor: Vedoucí bakalářské práce: Jakub Šindelka doc. PhDr. Marek Sedláček, Ph.D. Anotace Název práce: Analýza nejposlouchanějších skladeb na serveru YouTube.com Title: The analysis of the most listened compositions on YouTube.com Tato práce se zabývá analýzou devatenácti nejposlouchanějších hudebních skladeb na serveru YouTube.com, které byly uploadovány v roce 2014 a později. Seznam je aktuální k datu 1. 7. 2016, práce se tedy zabývá skladbami, které byly nejpopulárnější na serveru YouTube od začátku roku 2014 do poloviny roku 2016. U skladeb je zkoumán jejich žánr, délka, tempo, harmonie, struktura a další charakteristiky, práce se zabývá rovněž interprety těchto skladeb a jejich životem, tvorbou a uměleckou kariérou. Závěrečná část práce je věnována vyhodnocení toho, jaké znaky jsou u skladeb nejčastější a nejtypičtější. Okrajově se práce zabývá rovněž historií a principem fungování serveru YouTube. This thesis is focused on the analysis of the nineteen most listened music compositions on YouTube.com, which were uploaded in 2014 or later. The list is relevant to 1. 7. 2016, therefore the point of interest of the thesis are composions, which were the most popular in the period since the beginning of 2014 to the half of 2016. The aspects of compositions which are analyzed are their genre, lenght, tempo, harmony, structure and other characteristics, thesis also tries to comprehend the life, artworks and carrier of interprets of the compositions. The goal of the final part of this thesis is to evaluate which characteristics of the compositions are the most frequent and the most typical ones. -
New Ways of Being in the Fiction of Yoshimoto Banana
SINGLE FRAME HEROICS: NEW WAYS OF BEING IN THE FICTION OF YOSHIMOTO BANANA Ph. D Thesis Martin Ramsay Swinburne University of Technology 2009 CONTENTS Legend............................................................................................................. 5 Disclaimer…………………………………………………………………... 6 Acknowledgements…………………………………………………………. 7 Abstract ….…………………………………………………………………. 8 Introduction: A Literature of ‘Self-Help’………………………………… 9 Yoshimoto’s postmodern style…...………………………………………….. 11 Early success and a sense of impasse………………………………………... 15 A trans-cultural writer……………………………………………………….. 17 Rescuing literature from irrelevance………………………………………… 21 Chapter One: Women and Gender Roles in Contemporary Japanese Society………………………………………………………………………. 27 An historical overview ………………………………………….…………... 27 Nation building and changing ‘ideals of femininity’………………………... 30 The rise of the Modan Ga-ru (Modern Girl)………………………………… 32 The Post-War Experience ……………………………………….………….. 37 The emergence of the ‘parasite single’……………………………………… 38 Women’s magazines and changing ‘ideals of femininity’…………………... 41 The Women’s Liberation movement……………………………….………... 44 Fear of the young: The politics of falling birth rates……..………………….. 47 Chapter Two: Yoshimoto Banana and Contemporary Japanese Literature…....…………………………………………………………….. 53 Japanese literature, women and modernity …………………………………. 54 The problem with popular culture …………………………….…………….. 62 2 Sh ôjo culture: the ‘baby-doll face of feminism’ in Japan……..……………. 70 A global literature and a shared -
Pilot Project in Rural Western Madhya Pradesh, India, to Assess the Feasibility of Using LED And
View metadata, citation and similar papers at core.ac.uk brought to you by CORE JBUR-4452; No. of Pages 9 provided by Cronfa at Swansea University b u r n s x x x ( 2 0 1 4 ) x x x – x x x Available online at www.sciencedirect.com ScienceDirect journal homepage: www.elsevier.com/locate/burns Pilot project in rural western Madhya Pradesh, India, to assess the feasibility of using LED and solar-powered lanterns to remove kerosene lamps and related hazards from homes a, a a c b S. Chamania *, R. Chouhan , A. Awasthi , R. Bendell , N. Marsden , d b b J. Gibson , I.S. Whitaker , T.S. Potokar a Interburns Training Centre at Choithram Hospital, Indore, Madhya Pradesh, India b Welsh Centre for Burns and Plastic Surgery, Morriston Hospital, Swansea SA6 6NL, UK c Interburns, UK d University of Cardiff Medical School, Cardiff, UK a r t i c l e i n f o a b s t r a c t Article history: Background: Globally, 300,000 deaths are estimated to occur annually and the incidence is far Accepted 3 September 2014 greater as a large majority of burns are small and go unreported. Ninety-five percent of the global burden of burns is found in low- and middle-income countries; however, there is Keywords: relatively little in the literature regarding effective primary prevention in these areas. Flame is the most common cause of burn in Madhya Pradesh, the central state of India. The most Kerosene lamp common demographic among the burn unit inpatient of Choithram hospital Indore, is Kerosene burns young women from 21 to 40 years of age, whose burns are primarily caused by kerosene Sustainable lighting lamps. -
Sia - Elastic Heart Feat
antyteksty.com - teksty, które coś znaczą Dominika Bona Sia - Elastic Heart feat. Shia LaBeouf & Maddie Ziegler Sia - Elastic Heart feat. Shia LaBeouf & Maddie Ziegler „Elastic Heart” to utwór nagrany na potrzeby filmu „Igrzyska Śmierci: W pierścieniu ognia”. Teledysk przedstawia zamkniętą w klatce parę, która poprzez taniec stara się wyrazić swoje uczucia związane z rozstaniem. W role bohaterów wcielili sie 28-letni aktor Shia LaBeouf oraz 12-letnia tancerka Maddie Ziegler znana z klipu do „Chandelier”. Sia Kate Isobelle Furler, znana pod pseudonimem Sia, swoją karierę rozpoczęła śpiewając w zespole Crisp, z którym nagrała album “Only See”. Przed 2000 przeprowadziła się do Anglii, gdzie podpisała kontrakt z Dance Pool (sub-label Sony Music). W tym samym roku rozpoczęła karierę solową, wyda- jąc płytę „Healing Is Difficult”. Utwór „Taken For Granted”, zamieszczony w tym samym albumie,- znalazł się w pierwszej dziesiątce na liście angielskich przebojów. W 2004 podpisała kontrakt z Go!Beat Records. Wtedy też nagrała album „Colour the Small One”. Tekst piosenki Sia — Elastic Heart feat. Shia LaBe- Tłumaczenie Sia — Elastic Heart feat. Shia LaBe- ouf & Maddie Ziegler ouf & Maddie Ziegler And another one bites the dust I kolejna osoba gryzie ziemię Oh, why can I not conquer love Dlaczego nie mogę przezwyciężyć miłości And I might have thought that we were one A mogłam pomyśleć, że byliśmy jednością Wanted to fight this war without weapons Chciałam walczyć w tej wojnie bez broni And I wanted it, I wanted it bad Pragnęłam tego, bardzo chciałam -
Proquest Dissertations
Library and Archives Bibliotheque et l+M Canada Archives Canada Published Heritage Direction du Branch Patrimoine de I'edition 395 Wellington Street 395, rue Wellington Ottawa ON K1A 0N4 Ottawa ON K1A 0N4 Canada Canada Your file Votre reference ISBN: 978-0-494-54229-3 Our file Notre reference ISBN: 978-0-494-54229-3 NOTICE: AVIS: The author has granted a non L'auteur a accorde une licence non exclusive exclusive license allowing Library and permettant a la Bibliotheque et Archives Archives Canada to reproduce, Canada de reproduire, publier, archiver, publish, archive, preserve, conserve, sauvegarder, conserver, transmettre au public communicate to the public by par telecommunication ou par I'lnternet, preter, telecommunication or on the Internet, distribuer et vendre des theses partout dans le loan, distribute and sell theses monde, a des fins commerciales ou autres, sur worldwide, for commercial or non support microforme, papier, electronique et/ou commercial purposes, in microform, autres formats. paper, electronic and/or any other formats. The author retains copyright L'auteur conserve la propriete du droit d'auteur ownership and moral rights in this et des droits moraux qui protege cette these. Ni thesis. Neither the thesis nor la these ni des extraits substantiels de celle-ci substantial extracts from it may be ne doivent etre imprimes ou autrement printed or otherwise reproduced reproduits sans son autorisation. without the author's permission. In compliance with the Canadian Conformement a la loi canadienne sur la Privacy Act some supporting forms protection de la vie privee, quelques may have been removed from this formulaires secondaires ont ete enleves de thesis. -
Radical Healing: Restoring Hope in Urban Youth Through Afterschool Programming
Radical Healing: Restoring hope in urban youth through afterschool programming By MEGAN MUELLER B.S. (University of Minnesota) 2010 THESIS Submitted in partial satisfaction of the requirements for the degree of MASTER OF SCIENCE in Community Development in the OFFICE OF GRADUATE STUDIES of the UNIVERSITY OF CALIFORNIA DAVIS Approved: hello hope Sheryl-Ann Simpson, Chair how are you Natalia Deeb-Sossa finances Shawn A. Ginwright Committee in Charge 2017 i ProQuest Number:10286063 All rights reserved INFORMATION TO ALL USERS The quality of this reproduction is dependent upon the quality of the copy submitted. In the unlikely event that the author did not send a complete manuscript and there are missing pages, these will be noted. Also, if material had to be removed, a note will indicate the deletion. ProQuest 10286063 Published by ProQuest LLC ( 2017). Copyright of the Dissertation is held by the Author. All rights reserved. This work is protected against unauthorized copying under Title 17, United States Code Microform Edition © ProQuest LLC. ProQuest LLC. 789 East Eisenhower Parkway P.O. Box 1346 Ann Arbor, MI 48106 - 1346 ABSTRACT This research explores the alignment between Positive Youth Development (PYD) and the Radical Healing Framework (RHF), using Project Voice, an afterschool program for high school girls, as a case study. The RHF (Ginwright, 2016) is a tool used in youth programs and community organizations to address individual and collective trauma due to the systemic oppression experienced in urban, precarious (Butler, 2009) communities of color. This research evaluates the impact programming elements like action projects, healing rituals, and critical consciousness development have on the well-being of Project Voice participants and explores the potential afterschool programs have at restoring and sustaining public health. -
Pentecostal Music in the Public Square: the Christian Songs and Music of Juan Luis Guerra
Te Asbury Journal 72/1: 60-77 © 2017 Asbury Teological Seminary DOI: 10.7252/Journal.01.2017S.05 Kelly J. Godoy de Danielson and Robert Danielson Pentecostal Music in the Public Square: The Christian Songs and Music of Juan Luis Guerra Abstract This article explores the issue of contextualization of music in Latin America, particularly through the lens of Pentecostal singer-songwriter Juan Luis Guerra and his story of healing and conversion. Instead of leaving the pop music scene that had made him famous, he chose instead to stay in pop music and introduce Pentecostal Christian songs into his secular albums and concerts. This is a continuation of a long history of creative contextualization by Pentecostal musicians in sharp contrast to mainline Protestants who still primarily rely on translations of English hymns and music in a world where music is an integral part of the culture. Keywords: music, Pentecostalism, Juan Luis Guerra, contextualization, Latin America Kelly J. Godoy de Danielson is a M.A. in Biblical Studies student at Asbury Theological Seminary. She is a native of El Salvador, where she grew up and worked as an English-as-a-Second-Language teacher in the public schools. Her father worked in radio, and so she developed an interest in Latin American popular music and its role in Hispanic culture. She continues to be interested in how language intersects with theology, both in biblical languages and in modern languages in today’s context. Robert Danielson is an affliate professor at Asbury Theological Seminary and is the Scholarly Communications Librarian for the Seminary. -
Clinical and Experimental Studies in Idiopathic and Crohn's-Related Anal Fistula
Clinical and experimental studies in idiopathic and Crohn's-related anal fistula By Philip James Tozer MBBS MRCS Eng MCEM Thesis submitted for the degree of MD(Res) Department of Surgery and Cancer Imperial College London 2011 From The Fistula Research Unit, St Mark’s Hospital Watford Road, Harrow, HA1 3UJ Declaration of originality The following thesis and all studies embodied therein represent my own work including data collection and analysis, specimen retrieval, transport and processing of tissue, walk out, flow cytometry and other immunological techniques, histology, immunohistochemistry and fluorescent in situ hybridisation. Surgical procedures and colonoscopies were undertaken by the clinical staff at St Mark’s Hospital. Electron microscopy was kindly performed by the Centre for Ultrastructural Imaging at Kings College, London, and assistance in multiplex analysis was provided by the Antigen Presenting Research Group, Imperial College London. A proportion of the 3 year MRI data presented (up to 18 months follow up in around three quarters of the patients) were collected before I took over the running of the study. All other work reported in this thesis is appropriately referenced. 2 Abstract The factors leading to the creation and persistence of anal fistula in Crohn’s disease are poorly understood. As with luminal Crohn’s disease genetic, microbiological and immunological factors are implicated but the immunological and microbiological composition of Crohn’s and idiopathic anal fistulae have been obscure. My data demonstrate a lack of clinically relevant organisms within fistula tracts, a luminally driven immune response and subtle differences in this response between Crohn’s and idiopathic fistulae which may provide the basis for diagnostic tests, interventions and further research. -
Simplicity Two Thousand (M
ELECTRONICA_CLUBMUSIC_SOUNDTRACKS_&_MORE URBANI AfterlifeSimplicityTwoThousand AfterlifeSimplicityTwoThousand(mixes) AirTalkieWalkie Apollo440Gettin'HighOnYourOwnSupply ArchiveNoise ArchiveYouAllLookTheSameToMe AsianDubFoundationRafi'sRevenge BethGibbons&RustinManOutOfSeason BjorkDebut BjorkPost BjorkTelegram BoardsOfCanadaMusicHasTheRightToChildren DeathInVegasScorpioRising DeathInVegasTheContinoSessions DjKrushKakusei DjKrushReloadTheRemixCollection DjKrushZen DJShadowEndtroducing DjShadowThePrivatePress FaithlessOutrospective FightClub_stx FutureSoundOfLondonDeadCities GorillazGorillaz GotanProjectLaRevanchaDelTango HooverphonicBlueWonderPowerMilk HooverphonicJackieCane HooverphonicSitDownAndListenToH HooverphonicTheMagnificentTree KosheenResist Kruder&DorfmeisterConversionsAK&DSelection Kruder&DorfmeisterDjKicks LostHighway_stx MassiveAttack100thWindow MassiveAttackHits...BlueLine&Protection MaximHell'sKitchen Moby18 MobyHits MobyHotel PhotekModusOperandi PortisheadDummy PortisheadPortishead PortisheadRoselandNYCLive Portishead&SmokeCityRareRemixes ProdigyTheCastbreeder RequiemForADream_stx Spawn_stx StreetLifeOriginals_stx TerranovaCloseTheDoor TheCrystalMethodLegionOfBoom TheCrystalMethodLondonstx TheCrystalMethodTweekendRetail TheCrystalMethodVegas TheStreetsAGrandDon'tComeforFree ThieveryCorporationDJKicks(mix1999) ThieveryCorporationSoundsFromTheThieveryHiFi ToxicLoungeLowNoon TrickyAngelsWithDirtyFaces TrickyBlowback TrickyJuxtapose TrickyMaxinquaye TrickyNearlyGod TrickyPreMillenniumTension TrickyVulnerable URBANII 9Lazy9SweetJones -
An Evaluation of Environmental Goods (Egs) for the WTO EGA: Egs for Developing Countries
Project report An Evaluation of Environmental Goods (EGs) for the WTO EGA: EGs for Developing Countries Haley Knudson, Dina Margrethe Aspen and John Eilif Hermansen Trondheim, 16 January, 2015 1 NTNU Trondheim Postadresse: 7491 Trondheim Norges teknisk-naturvitenskapelige universitet Besøksadresse: Alfred Getz vei 1 Fakultet for samfunnsvitenskap og teknologiledelse Telefon: 73 59 35 11 Institutt for industriell økonomi og teknologiledelse Telefaks 73 59 10 45 Org.nr. 974 767 880 Title: Report no.: - - An Evaluation of Environmental Goods (EGs) for the WTO EGA: EGs for Developing Countries Project: Project no.: 3104110 Consultancy Assistance Agreement Contract nr. 14/9951 Contracting partners: Date: 16.01.2015 Royal Norwegian Ministry of Foreign Affairs and NTNU, Department of Industrial Economics and Number of pages: 104 Technology Management (IØT) Number of appendices: 2 Authors: Signature: Haley Knudson, Dina Magrethe Aspen and John Eilif Hermansen Haley Knudson Responsible: Signature: Department of Industrial Economics and Technology Management (IOT), John Eilif Hermansen Norwegian University of Science and Technology (NTNU) Project leader: John E Hermansen Summary: The paper presents the design, analyses and results of a methodological assessment and nomination of goods for the ongoing WTO Environmental Goods Agreement (EGA) negotiations. The purpose of the EGA is to reduce tariffs on identified environmental goods and technologies in order to promote international sustainable production, consumption and development. The methodology presented is based on multi criteria decision making (MCDM) tools and management. The Norwegian Government’s focus on supporting the developing world has guided their international policy over the past years. This study is therefore commissioned by the Norwegian Ministry of Foreign affairs to ensure that goods of particular relevance to developing countries will be discussed in the EGA. -
Adopting Multifunctional Material Systems
ADOPTING MULTIFUNCTIONAL MATERIAL SYSTEMS by ANDRÉ DUARTE B. L. FERREIRA A Dissertation Presented to the Faculty of Engineering of the University of Oporto In partial fulfillment of the requirements for the Degree of MASTER OF SCIENCE IN MECHANICAL ENGINEERING OPORTO, JULY 2015 Adopting Multifunctional Material Systems ii Adopting Multifunctional Material Systems I hereby declare that all information in this document has been obtained and presented in accordance with academic rules and ethical conduct. I also declare that, as required by these rules and conduct, I have fully cited and referenced all material and results that are not original to this work. Name, Last Name: ANDRÉ, FERREIRA Signature_______________________________________ iii Adopting Multifunctional Material Systems ABSTRACT ADOPTING MULTIFUNCTIONAL MATERIAL SYSTEMS Ferreira, André Duarte M.S., Department of Mechanical Engineering Supervisor: Prof. Dr. A.T. Marques July 2015, 174 pages An increase in system-level efficiency and polyvalence of products and components is one of the main advantages of the use of some novel polyvalent materials and structures. This polyvalence or multifunctionality is currently a hot topic in science and engineering circles and is gathering increasingly more attention. Presently this multifunctionality can be achieved by stimuli responsive materials such as temperature, stress and light, by using shape memory materials, by using surface structures with anti-biofouling and drag reduction capabilities, among many others. In this dissertation, an overview is pre- sented of the topic, including state-of-the-art review, challenges, suggestions and pro- posed methods to overcome them and to increase innovation in developing both these materials and structures and the products that contain them. -
American Eagle Outfitters Launches Music Program for New Sub-Brand Aerie
NEWS RELEASE American Eagle Outfitters Launches Music Program for New Sub-Brand aerie 9/6/2006 aerie Artists Music Series Features Free Exclusive Downloadable Tracks and Videos, In-Store Concerts and Special-Edition CDs NEW YORK, Sept. 6 /PRNewswire-FirstCall/ -- American Eagle Outfitters (Nasdaq: AEOS), today announced a new music program for aerie customers, the aerie Artists Music Series. aerie by American Eagle is a new line of intimates and dormwear, which is making its official debut in AE stores today. The aerie Artists Music Series identifies emerging and independent musicians whose work reflects and inspires the aerie girl's lifestyle. The Series will premier exclusive tracks and music videos on www.aerie.com, offering them to customers for download free-of- charge. Recording artists Ben Lee and Sia are the first to be selected for the aerie Artists Music Series, which today released the world premier of Lee's single, "Since I met you" and Sia's single, "Pictures." Both songs are available as free exclusive downloads on www.aerie.com. Lee will also perform live at the American Eagle Union Square Store in New York City tonight. "The aerie Artists Music Series is about helping our customers discover music they'll love as part of their aerie shopping experience," said Kathy Savitt, chief marketing officer of American Eagle Outfitters. "We're thrilled to launch the Series with such talented and inspiring artists like Ben Lee and Sia." The aerie Artists Music Series will also release a special edition of Ben Lee's CD "Awake is the New Sleep," which 1 includes the exclusive aerie single, "Since I Met You" and artist interviews.