Phlpping Through History: a Decade in the Life of PHLPP Phosphatases
Total Page:16
File Type:pdf, Size:1020Kb
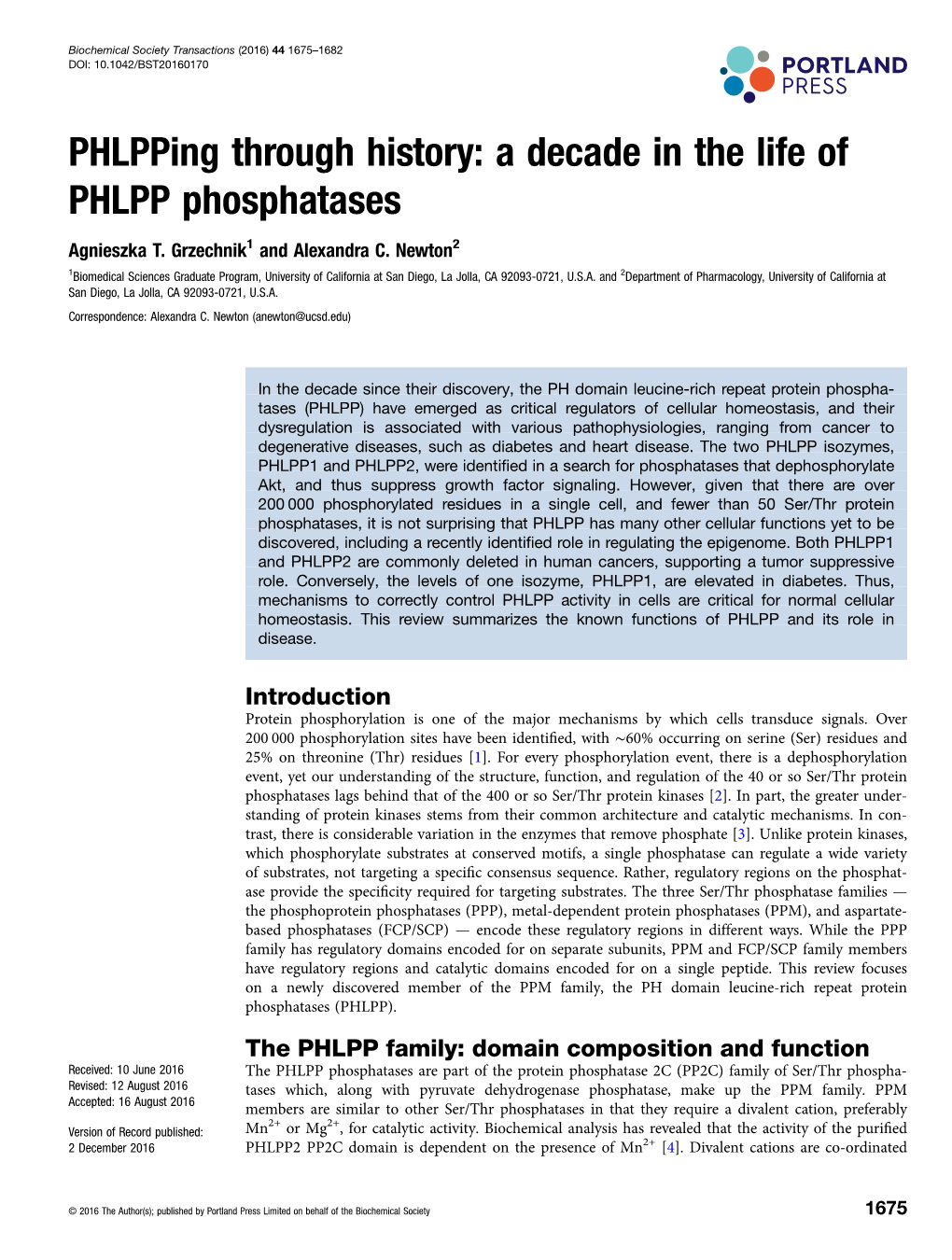
Load more
Recommended publications
-
The Regulatory Roles of Phosphatases in Cancer
Oncogene (2014) 33, 939–953 & 2014 Macmillan Publishers Limited All rights reserved 0950-9232/14 www.nature.com/onc REVIEW The regulatory roles of phosphatases in cancer J Stebbing1, LC Lit1, H Zhang, RS Darrington, O Melaiu, B Rudraraju and G Giamas The relevance of potentially reversible post-translational modifications required for controlling cellular processes in cancer is one of the most thriving arenas of cellular and molecular biology. Any alteration in the balanced equilibrium between kinases and phosphatases may result in development and progression of various diseases, including different types of cancer, though phosphatases are relatively under-studied. Loss of phosphatases such as PTEN (phosphatase and tensin homologue deleted on chromosome 10), a known tumour suppressor, across tumour types lends credence to the development of phosphatidylinositol 3--kinase inhibitors alongside the use of phosphatase expression as a biomarker, though phase 3 trial data are lacking. In this review, we give an updated report on phosphatase dysregulation linked to organ-specific malignancies. Oncogene (2014) 33, 939–953; doi:10.1038/onc.2013.80; published online 18 March 2013 Keywords: cancer; phosphatases; solid tumours GASTROINTESTINAL MALIGNANCIES abs in sera were significantly associated with poor survival in Oesophageal cancer advanced ESCC, suggesting that they may have a clinical utility in Loss of PTEN (phosphatase and tensin homologue deleted on ESCC screening and diagnosis.5 chromosome 10) expression in oesophageal cancer is frequent, Cao et al.6 investigated the role of protein tyrosine phosphatase, among other gene alterations characterizing this disease. Zhou non-receptor type 12 (PTPN12) in ESCC and showed that PTPN12 et al.1 found that overexpression of PTEN suppresses growth and protein expression is higher in normal para-cancerous tissues than induces apoptosis in oesophageal cancer cell lines, through in 20 ESCC tissues. -
Antigen-Specific Memory CD4 T Cells Coordinated Changes in DNA
Downloaded from http://www.jimmunol.org/ by guest on September 24, 2021 is online at: average * The Journal of Immunology The Journal of Immunology published online 18 March 2013 from submission to initial decision 4 weeks from acceptance to publication http://www.jimmunol.org/content/early/2013/03/17/jimmun ol.1202267 Coordinated Changes in DNA Methylation in Antigen-Specific Memory CD4 T Cells Shin-ichi Hashimoto, Katsumi Ogoshi, Atsushi Sasaki, Jun Abe, Wei Qu, Yoichiro Nakatani, Budrul Ahsan, Kenshiro Oshima, Francis H. W. Shand, Akio Ametani, Yutaka Suzuki, Shuichi Kaneko, Takashi Wada, Masahira Hattori, Sumio Sugano, Shinichi Morishita and Kouji Matsushima J Immunol Submit online. Every submission reviewed by practicing scientists ? is published twice each month by Author Choice option Receive free email-alerts when new articles cite this article. Sign up at: http://jimmunol.org/alerts http://jimmunol.org/subscription Submit copyright permission requests at: http://www.aai.org/About/Publications/JI/copyright.html Freely available online through http://www.jimmunol.org/content/suppl/2013/03/18/jimmunol.120226 7.DC1 Information about subscribing to The JI No Triage! Fast Publication! Rapid Reviews! 30 days* Why • • • Material Permissions Email Alerts Subscription Author Choice Supplementary The Journal of Immunology The American Association of Immunologists, Inc., 1451 Rockville Pike, Suite 650, Rockville, MD 20852 Copyright © 2013 by The American Association of Immunologists, Inc. All rights reserved. Print ISSN: 0022-1767 Online ISSN: 1550-6606. This information is current as of September 24, 2021. Published March 18, 2013, doi:10.4049/jimmunol.1202267 The Journal of Immunology Coordinated Changes in DNA Methylation in Antigen-Specific Memory CD4 T Cells Shin-ichi Hashimoto,*,†,‡ Katsumi Ogoshi,* Atsushi Sasaki,† Jun Abe,* Wei Qu,† Yoichiro Nakatani,† Budrul Ahsan,x Kenshiro Oshima,† Francis H. -
Emerging Roles of PHLPP Phosphatases in Cell Signaling
PA61CH26_Newton ARjats.cls September 22, 2020 12:5 Annual Review of Pharmacology and Toxicology PHLPPing the Script: Emerging Roles of PHLPP Phosphatases in Cell Signaling Timothy R. Baffi,∗ Ksenya Cohen Katsenelson,∗ and Alexandra C. Newton Department of Pharmacology, University of California, San Diego, La Jolla, California 92093-0721, USA; email: [email protected] Annu. Rev. Pharmacol. Toxicol.2021. 61:26.1–26.21 Keywords The Annual Review of Pharmacology and Toxicology is PHLPP, Akt, PKC, phosphatase, phosphorylation, cancer, transcription online at pharmtox.annualreviews.org https://doi.org/10.1146/annurev-pharmtox-031820- Abstract 122108 Whereas protein kinases have been successfully targeted for a variety of dis- Copyright © 2021 by Annual Reviews. eases, protein phosphatases remain an underutilized therapeutic target, in All rights reserved part because of incomplete characterization of their effects on signaling net- ∗ These authors contributed equally to this article works. The pleckstrin homology domain leucine-rich repeat protein phos- Annu. Rev. Pharmacol. Toxicol. 2021.61. Downloaded from www.annualreviews.org phatase (PHLPP) is a relatively new player in the cell signaling field, and new Access provided by University of California - San Diego on 11/11/20. For personal use only. roles in controlling the balance among cell survival, proliferation, and apop- tosis are being increasingly identified. Originally characterized for its tumor- suppressive function in deactivating the prosurvival kinase Akt, PHLPP may have an opposing role in promoting survival, as recent evidence suggests. Additionally, identification of the transcription factor STAT1 as a substrate unveils a role for PHLPP as a critical mediator of transcriptional programs in cancer and the inflammatory response. -
Kidney V-Atpase-Rich Cell Proteome Database
A comprehensive list of the proteins that are expressed in V-ATPase-rich cells harvested from the kidneys based on the isolation by enzymatic digestion and fluorescence-activated cell sorting (FACS) from transgenic B1-EGFP mice, which express EGFP under the control of the promoter of the V-ATPase-B1 subunit. In these mice, type A and B intercalated cells and connecting segment principal cells of the kidney express EGFP. The protein identification was performed by LC-MS/MS using an LTQ tandem mass spectrometer (Thermo Fisher Scientific). For questions or comments please contact Sylvie Breton ([email protected]) or Mark A. Knepper ([email protected]). -
Papadaki Et Al., 2009 Supplementary
Papadaki et al., 2009 Supplementary Supplemental Data Index x Supplemental Figures 1-6 x Supplemental Tables 1a, 1b, 2 Papadaki et al., 2009 Supplementary Supplemental Figure 1. Thymocyte restricted inactivation of the Elavl1 locus. + fl (A) Diagrammatic representation of the wild-type (Elavl1P P), floxed (Elavl1P P) and Cre- - recombined (Elavl1P P) Elavl1/HuR loci on mouse chromosome 8; Noted are the loxP sequences (triangles) flanking the selection marker (neo) used in gene targeting and the ATG containing exon 2 (white box); (H) denotes restriction sites for loci mapping. (B) Detection of native (+), targeted (fl) and Cre-recombinant (-) loci in thymocyte DNA extracts from control and test mice following HindIII digestion and Southern blotting. (C) Western blot of total thymic protein extracts probed with ĮHuR Ab + fl/fl indicating the loss of HuR protein in LckCreP PElavl1P P thymi. Į-actin is shown for quantitation. (D) Flow cytometric detection of intracellular mHuR protein in + fl/+ LckCreP PElavl1P P thymocytes (open histogram), and its respective loss in + fl/fl LckCreP PElavl1P P thymocytes (shaded histogram). The dotted histogram depicts the + isotype-matched background staining. (E) Flow cytometric detection of HuRP P or - + + + fl/+ HuRP P cells in gated splenic CD4P Por CD8P P T-cells from 8 week old LckCreP PElavl1P + fl/fl - Pand LckCreP PElavl1P P mice respectively. (F) Enumeration of HuRP P cells in + fl/fl LckCreP PElavl1P P thymocyte subsets and splenic T-cells; Data are percentages (+SEM) derived from the flow cytometric detection of HuR- cells in CD4/CD8/DP and DN gated populations (n=12-15) at 8-10 weeks of age. -
MTOR Signaling and Metabolism in Early T Cell Development
G C A T T A C G G C A T genes Review MTOR Signaling and Metabolism in Early T Cell Development Guy Werlen, Ritika Jain and Estela Jacinto * Department of Biochemistry and Molecular Biology, Robert Wood Johnson Medical School, Rutgers University, Piscataway, NJ 08854, USA; [email protected] (G.W.); [email protected] (R.J.) * Correspondence: [email protected]; Tel.: +1-732-235-4476 Abstract: The mechanistic target of rapamycin (mTOR) controls cell fate and responses via its func- tions in regulating metabolism. Its role in controlling immunity was unraveled by early studies on the immunosuppressive properties of rapamycin. Recent studies have provided insights on how metabolic reprogramming and mTOR signaling impact peripheral T cell activation and fate. The contribution of mTOR and metabolism during early T-cell development in the thymus is also emerging and is the subject of this review. Two major T lineages with distinct immune functions and peripheral homing organs diverge during early thymic development; the αβ- and γδ-T cells, which are defined by their respective TCR subunits. Thymic T-regulatory cells, which have immunosup- pressive functions, also develop in the thymus from positively selected αβ-T cells. Here, we review recent findings on how the two mTOR protein complexes, mTORC1 and mTORC2, and the signaling molecules involved in the mTOR pathway are involved in thymocyte differentiation. We discuss emerging views on how metabolic remodeling impacts early T cell development and how this can be mediated via mTOR signaling. Keywords: mTOR; mTORC1; mTORC2; thymocytes; T lymphocytes; early T cell development; T-cell metabolism Citation: Werlen, G.; Jain, R.; Jacinto, E. -
Foxo Transcription Factors Activate Akt and Attenuate Insulin Signaling in Heart by Inhibiting Protein Phosphatases
FoxO transcription factors activate Akt and attenuate insulin signaling in heart by inhibiting protein phosphatases Yan G. Ni*†, Na Wang*, Dian J. Cao*, Nita Sachan*, David J. Morris*, Robert D. Gerard*‡, Makoto Kuro-o§, Beverly A. Rothermel*, and Joseph A. Hill*‡¶ʈ ¶Donald W. Reynolds Cardiovascular Clinical Research Center, Departments of *Internal Medicine (Cardiology), ‡Molecular Biology, and §Pathology, University of Texas Southwestern Medical Center, Dallas, TX 75390-8573 Edited by Eric N. Olson, University of Texas Southwestern Medical Center, Dallas, TX, and approved November 7, 2007 (received for review November 21, 2006) Insulin resistance and metabolic syndrome are rapidly expanding The O subfamily of Forkhead/winged helix transcription factors public health problems. Acting through the PI3K/Akt pathway, (FoxO) plays important roles in regulating cardiac and skeletal insulin and insulin-like growth factor-1 (IGF-1) inactivate FoxO muscle remodeling (8–11). FoxO proteins are phylogenetically transcription factors, a class of highly conserved proteins impor- conserved and regulate key physiological functions, including cell tant in numerous physiological functions. However, even as FoxO proliferation, cell differentiation, and survival (12–15). In addition, is a downstream target of insulin, FoxO factors also control FoxO functions in complex ways to regulate insulin signaling and upstream signaling elements governing insulin sensitivity and glucose and lipid metabolism (13, 16). In Drosophila, activation of glucose metabolism. Here, we report that sustained activation of dFoxO in fat body represses insulin-dependent signaling and in- either FoxO1 or FoxO3 in cardiac myocytes increases basal levels of creases life span (17). Similarly in mammals, FoxO1 regulates Akt phosphorylation and kinase activity. -
Post-Translational Modifications and Stress Adaptation: the Paradigm of FKBP51
Biochemical Society Transactions (2020) 48 441–449 https://doi.org/10.1042/BST20190332 Review Article Post-translational modifications and stress adaptation: the paradigm of FKBP51 Theo Rein Max Planck Institute of Psychiatry, Department of Translational Research in Psychiatry, Munich, Germany Correspondence: Theo Rein ([email protected]) Adaptation to stress is a fundamental requirement to cope with changing environmental conditions that pose a threat to the homeostasis of cells and organisms. Post-transla- tional modifications (PTMs) of proteins represent a possibility to quickly produce proteins with new features demanding relatively little cellular resources. FK506 binding protein (FKBP) 51 is a pivotal stress protein that is involved in the regulation of several executers of PTMs. This mini-review discusses the role of FKBP51 in the function of proteins responsible for setting the phosphorylation, ubiquitination and lipidation of other proteins. Examples include the kinases Akt1, CDK5 and GSK3β, the phosphatases calcineurin, PP2A and PHLPP, and the ubiquitin E3-ligase SKP2. The impact of FKBP51 on PTMs of signal transduction proteins significantly extends the functional versatility of this protein. As a stress-induced protein, FKBP51 uses re-setting of PTMs to relay the effect of stress on various signaling pathways. Introduction The physiological stress response is elicited whenever a change in the environment is sensed and interpreted as threat to homeostasis. Effector systems comprise the autonomic nervous system and the hypothalamic-pituitary-adrenocortical (HPA) axis [1]. The effector molecules of these systems are noradrenaline and adrenaline for the autonomic nervous system, and cortisol for the HPA axis (corticosterone in rodents). Adrenaline and noradrenaline act through G protein-coupled receptors that are hooked to various intracellular pathways involving determinants of post-translational modifi- cations (PTMs) such as kinases and phosphatases [2]. -
Supplementary Table S1: Search Terms
Supplementary Table S1: Search Terms Gene Search Terms ACE Angiotensin I Converting Enzyme Angiotensin I Converting Enzyme (Peptidyl-Dipeptidase A) 1 Dipeptidyl Carboxypeptidase I CD143 Antigen Kininase II DCP1 DCP Angiotensin I Converting Enzyme Peptidyl-Dipeptidase A 1 Transcript Angiotensin Converting Enzyme, Somatic Isoform Peptidyl-Dipeptidase A Carboxycathepsin Testicular ECA Peptidase P EC 3.4.15.1 EC 3.2.1.- CD143 MVCD3 ACE1 ACE ICH AND Kidney Nephrology Nephropathy Renal AND SNP Polymorphism Variant Allele Genotype ACE2 Angiotensin I Converting Enzyme 2 Angiotensin I Converting Enzyme (Peptidyl-Dipeptidase A) 2 Angiotensin-Converting Enzyme Homolog ACE-Related Carboxypeptidase Metalloprotease MPROT15 Peptidyl-Dipeptidase A ACEH EC 3.4.17.23 EC 3.4.17 ACE2 AND Kidney Nephrology Nephropathy Renal AND SNP Polymorphism Variant Allele Genotype AGT Angiotensinogen Angiotensinogen (Serpin Peptidase Inhibitor, Clade A, Member 8) Serpin Peptidase Inhibitor, Clade A, Member 8 Serpin A8 SERPINA8 Serine (Or Cysteine) Proteinase Inhibitor Alpha-1 Antiproteinase, Antitrypsin Alpha-1 Antiproteinase Pre-Angiotensinogen Angiotensin II Angiotensin I Antitrypsin ANHU AGT AND Kidney Nephrology Nephropathy Renal AND SNP Polymorphism Variant Allele Genotype AGTR1 Angiotensin II Receptor Type 1 Angiotensin II Receptor, Type 1 AGTR1B AT1AR AT1BR AT2R1 AT1 Type-1B Angiotensin II Receptor Angiotensin II Type-1 Receptor Angiotensin Receptor 1B AGTR1A AT2R1B HAT1R AG2S AT1B AT1R AGTR1 AT2R1A AND Kidney Nephrology Nephropathy Renal AND SNP Polymorphism Variant -
Phosphorylation of NHERF1 S279 and S301 Differentially Regulates
BBA - Molecular Basis of Disease 1865 (2019) 26–37 Contents lists available at ScienceDirect BBA - Molecular Basis of Disease journal homepage: www.elsevier.com/locate/bbadis Phosphorylation of NHERF1 S279 and S301 differentially regulates breast T cancer cell phenotype and metastatic organotropism Maria Raffaella Grecoa,1, Emeline Bonb,1, Rosa Rubinoa, Lorenzo Guerraa, Manuel Bernabe-Garciac, Stefania Cannonea, Maria-Luisa Cayuelac, Loredana Ciacciaa, Séverine Marionneau-Lambotd, Thibauld Oullierd, Gaëlle Fromontb,e, Roseline Guibonb,e, ⁎ ⁎⁎ Sébastien Rogerb,f, ,2, Stephan Joel Reshkina, ,2, Rosa Angela Cardonea,2 a Department of Bioscience, Biotechnology and Biopharmaceutics, University of Bari, Italy b Université de Tours, Inserm UMR1069, Nutrition, Croissance et Cancer, Tours, France c Telomerase, Cancer and Aging Group, Research Unit, Department of Surgery, CIBERehd - University Hospital “Virgen de la Arrixaca”, Murcia, Spain d Cancéropôle du Grand Ouest, Nantes, France e Centre Hospitalo-Universitaire de Tours, Tours, France f Institut Universitaire de France, Paris, France ARTICLE INFO ABSTRACT Keywords: Metastatic cancer cells are highly plastic for the expression of different tumor phenotype hallmarks and orga- EBP50 notropism. This plasticity is highly regulated but the dynamics of the signaling processes orchestrating the shift SLC9A3R1 from one cell phenotype and metastatic organ pattern to another are still largely unknown. The scaffolding Invasion protein NHERF1 has been shown to regulate the expression of different neoplastic phenotypes through its PDZ Invadopodia domains, which forms the mechanistic basis for metastatic organotropism. This reprogramming activity was Metastases postulated to be dependent on its differential phosphorylation patterns. Here, we show that NHERF1 phos- Mesenchymal-vasculogenic transition Vasculogenic mimicry phorylation on S279/S301 dictates several tumor phenotypes such as in vivo invasion, NHE1-mediated matrix digestion, growth and vasculogenic mimicry. -
Pleckstrin Homology Domain Leucine-Rich Repeat Protein the Histone Code to Control the Transcription of Rtks
Pleckstrin homology domain leucine-rich repeat PNAS PLUS protein phosphatases set the amplitude of receptor tyrosine kinase output Gloria Reyesa,1, Matt Niedersta,b,1, Ksenya Cohen-Katsenelsona, Joshua D. Stenderc, Maya T. Kunkela, Muhan Chend, John Brognarda,2, Emma Siereckia,3, Tianyan Gaoe, Dawid G. Nowakc, Lloyd C. Trotmand, Christopher K. Glassc, and Alexandra C. Newtona,4 Departments of aPharmacology and cCellular and Molecular Medicine and bBiomedical Sciences Graduate Program, University of California, San Diego, La Jolla, CA 92093; dCold Spring Harbor Laboratory, Cold Spring Harbor, NY 11724; and eDepartment of Molecular and Cellular Biochemistry, University of Kentucky, Lexington, KY 40536 Edited by Tony Hunter, The Salk Institute for Biological Studies, La Jolla, CA, and approved August 14, 2014 (received for review March 6, 2014) Growth factor receptor levels are aberrantly high in diverse cancers, (18). Collaboration between phosphorylation and acetylation/ driving the proliferation and survival of tumor cells. Understanding methylation on histone tails influences a multitude of cellular the molecular basis for this aberrant elevation has profound clinical processes, including transcription of target genes. For example, implications. Here we show that the pleckstrin homology domain multiple lines of evidence support synergism between histone leucine-rich repeat protein phosphatase (PHLPP) suppresses receptor acetylation and phosphorylation in the induction of immediate- tyrosine kinase (RTK) signaling output by a previously unidentified early genes (such as c-jun, c-fos,andc-myc)aftermitogenicstimu- epigenetic mechanism unrelated to its previously described function lation. Furthermore, having one modification can increase the ef- as the hydrophobic motif phosphatase for the protein kinase AKT, ficiency of an enzyme catalyzing a second modification; for example, protein kinase C, and S6 kinase. -
The Glycolysis Regulator PFKFB4 Interacts with ICMT and Activates RAS/AKT Signaling-Dependent Cell Migration in Melanoma
bioRxiv preprint doi: https://doi.org/10.1101/2020.03.23.004119; this version posted March 24, 2020. The copyright holder for this preprint (which was not certified by peer review) is the author/funder. All rights reserved. No reuse allowed without permission. The glycolysis regulator PFKFB4 interacts with ICMT and activates RAS/AKT signaling-dependent cell migration in melanoma. Méghane Sittewelle1,2, Déborah Lécuyer1,2, Anne H. Monsoro-Burq1,2,3* 1Université Paris Saclay, CNRS UMR 3347, INSERM U1021, Centre Universitaire, 15, rue Georges Clémenceau, F-91405 Orsay, France 2Institut Curie Research Division, PSL Research University, CNRS UMR 3347, INSERM U1021, Rue Henri Becquerel, F-91405 Orsay, France 3Institut Universitaire de France, F-75005 Paris, France * author for correspondence: e-mail: [email protected] Key words. RAS signaling, AKT signaling, ICMT, PFKFB4, RAS localiZation, melanoma, cell migration 1 bioRxiv preprint doi: https://doi.org/10.1101/2020.03.23.004119; this version posted March 24, 2020. The copyright holder for this preprint (which was not certified by peer review) is the author/funder. All rights reserved. No reuse allowed without permission. Abstract. Cell migration is a complex process, tightly regulated during embryonic development and abnormally activated during cancer metastasis. RAS-dependent signaling is a major nexus controlling essential cell parameters such as proliferation, survival and migration using downstream effectors among which the PI3K/AKT signaling. In melanoma, oncogenic mutations frequently enhance RAS, PI3K/AKT or MAP kinase signaling, in addition to other cancer hallmarks including the activation of metabolism regulators such as PFKFB4, a critical regulator of glycolysis and Warburg effect.