Understanding Deformation with High Angular Resolution Electron
Total Page:16
File Type:pdf, Size:1020Kb
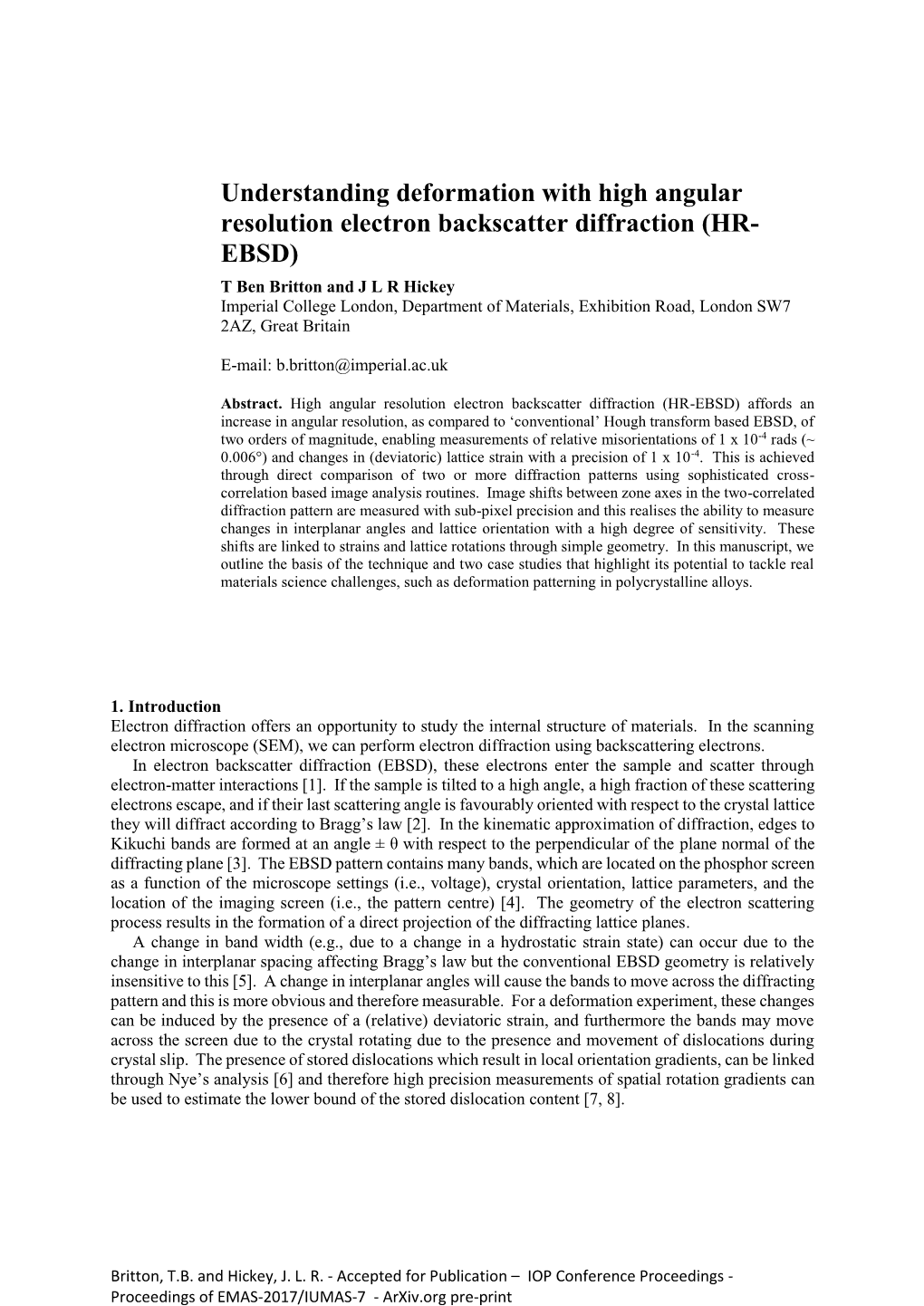
Load more
Recommended publications
-
Centre for Advanced Structural Ceramics 2019 Annual Report
Centre for Advanced Structural Ceramics 2019 Annual Report CASC Annual Report 2019 Introduction CASC has been continuously running since it started in July 2008 with EPSRC funding (£5.5M) for a five-year programme. The EPSRC funding came to an end in June 2013, but we are growing each year, establishing new industrial and academic collaborations both abroad and in the UK and participating in numerous national and international initiatives. This year a large number of PhD students have started working with us on a wide range of research projects, from biomimetic composites to transpiration cooling. Teaching and training is a big part of what we do. Our Ceramics Summer School is still going strong and we continue working in the organization of meetings and symposiums. The centre collaborates with Industry in several projects and maintains an Industrial Consortium to enable its sustainability and continue long-term and fruitful relationships between CASC’s associated academics and the UK’s ceramics community. Our main goal is to continue these relationships and grow as a ceramics centre. Congratulations also to Prof. Luc Vandeperre, winner of the 2019 IOM3 Verulam Medal & Prize Professor Eduardo Saiz CASC Director 2 Management CASC was initially set up by Professor Bill Lee in 2008. In 2012, he was succeeded as Director by Professor Eduardo Saiz. Local Management Team (LMT) The LMT is responsible for managing the centre’s operations and meets the second Thursday of every month to oversee the pressing day-to-day issues of running the Centre. These issues include staff appointments, equipment purchase, finances and building refurbishment, but are increasingly focussed on developing the Centre national and international profile, forging industrial links and achieving financial sustainability. -
Nano-Scale Behavior of Irradiated Nano-Structured Alloys David Armstrong University of Oxford, [email protected]
Engineering Conferences International ECI Digital Archives Nanomechanical Testing in Materials Research and Proceedings Development V Fall 10-8-2015 Nano-scale behavior of irradiated nano-structured alloys David Armstrong University of Oxford, [email protected] John Waite University of Oxford Steve Fitzgerald University of Oxford Angus Wilkinson University of Oxford Alan Xu University of Oxford See next page for additional authors Follow this and additional works at: http://dc.engconfintl.org/nanomechtest_v Part of the Materials Science and Engineering Commons Recommended Citation David Armstrong, John Waite, Steve Fitzgerald, Angus Wilkinson, Alan Xu, and T. Ben Britton, "Nano-scale behavior of irradiated nano-structured alloys" in "Nanomechanical Testing in Materials Research and Development V", Dr. Marc Legros, CEMES-CNRS, France Eds, ECI Symposium Series, (2015). http://dc.engconfintl.org/nanomechtest_v/48 This Abstract is brought to you for free and open access by the Proceedings at ECI Digital Archives. It has been accepted for inclusion in Nanomechanical Testing in Materials Research and Development V by an authorized administrator of ECI Digital Archives. For more information, please contact [email protected]. Authors David Armstrong, John Waite, Steve Fitzgerald, Angus Wilkinson, Alan Xu, and T. Ben Britton This abstract is available at ECI Digital Archives: http://dc.engconfintl.org/nanomechtest_v/48 NANO-SCALE BEHAVIOR OF IRRADIATED NANOSTRUCTURED ALLOYS David E.J. Armstrong, Department of Materials, University of Oxford, UK [email protected] John Waite, Department of Materials, University of Oxford, UK Steve Fitzgerald, Department of Materials, University of Oxford, UK Angus Wilkinson, Department of Materials, University of Oxford, UK Alan, Xu, Department of Materials, University of Oxford, UK T. -
SPARC Adding Value to Additive Manufacturing-Advanced
SPARC Webex Seminar Adding Value To Additive Manufacturing-Advanced Characterization Of The Structure-Properties- Performance Of Ti6Al4V By Department of Materials, Imperial College, London, U. K. Department of Metallurgical and Materials Engineering, IIT Roorkee, India Department of Materials Science and Engineering, IIT Kanpur, India Talk 1 Tuesday 23 June 2020 3:30 pm IST 11 am BST Talk 2 Friday 26 June 2020 3:30 pm IST 11 am BST Talk 1: Understanding Deformation in the Scanning Electron Microscope Dr Ben Britton, Department of Materials, Imperial College London Abstract: In this talk, we will explore methods to understand deformation in the scanning electron microscope. This will include an introduction to electron channeling contrast imaging (ECCI) which can be used to image dislocations; the used of electron backscatter diffraction (EBSD), as well as High Angular Resolution (HR) EBSD, to understand lattice strain and stored dislocation content; and finally we will briefly reveal what can be observed using high spatial resolution digital image correlation (HR-DIC). Together with these introductions, we will explore a few case studies of how these can be used to understand the performance of alloys in demanding environments. Bio: Ben Britton leads the experimental micromechanics group, who focus on developing methods to characterise materials and understand how materials deform. The team have been developing new microscopy approaches, including EBSD techniques, as well as micromechanical testing techniques. These approaches have been applied to understand metals (Ni-, Ti-, Zr-, Fe-, Cu- based alloys) used in aerospace, oil & gas, and nuclear power applications. Their work can be explored at www.expmicromech.com. -
Kikuchi Pattern Spectrum
Constraints on the Effective Electron Energy Spectrum in Backscatter Kikuchi Diffraction Aimo Winkelmann,1, ∗ T. Ben Britton,2, y and Gert Nolze3 1Laser Zentrum Hannover e.V., Hollerithallee 8, 30419 Hannover, Germany 2Department of Materials, Imperial College London, London, UK 3Federal Institute for Materials, Research and Testing (BAM), Unter den Eichen 87, 12205 Berlin, Germanyz (Dated: October 24, 2018) Abstract Electron Backscatter Diffraction (EBSD) is a technique to obtain microcrystallographic informa- tion from materials by collecting large-angle Kikuchi patterns in the scanning electron microscope (SEM). An important fundamental question concerns the scattering-angle dependent electron en- ergy distribution which is relevant for the formation of the Kikuchi diffraction patterns. Here we review the existing experimental data and explore the effective energy spectrum that is operative in the generation of backscatter Kikuchi patterns from silicon. We use a full pattern compari- son of experimental data with dynamical electron diffraction simulations. Our energy-dependent cross-correlation based pattern matching approach establishes improved constraints on the effective Kikuchi pattern energy spectrum which is relevant for high-resolution EBSD pattern simulations and their applications. arXiv:1810.09525v1 [cond-mat.mtrl-sci] 22 Oct 2018 ∗ [email protected] y [email protected] z [email protected] 1 a FIG. 1. EBSD scattering geometry and raw diffraction pattern with key features. For a specific point on the phosphor screen, the angle α indicates the scattering angle relative to the primary ◦ ◦ beam, with 30 / α / 130 for typical EBSD patterns. I. INTRODUCTION Electron backscatter diffraction (EBSD) is a technique which is used to reveal the mi- crostructure of crystalline materials, including metals, ceramics, functional materials, and minerals in the scanning electron microscope (SEM) [1]. -
Mechanical and Microstructural Investigations of Tungsten and Doped Tungsten Materials Produced Via Powder Injection Molding
Nuclear Materials and Energy 3–4 (2015) 22–31 Contents lists available at ScienceDirect Nuclear Materials and Energy journal homepage: www.elsevier.com/locate/nme Mechanical and microstructural investigations of tungsten and doped tungsten materials produced via powder injection molding Steffen Antusch a,∗, David E.J. Armstrong b, T. Ben Britton c, Lorelei Commin a, James S.K.-L. Gibson b, Henri Greuner d,JanHoffmanna, Wolfram Knabl e, Gerald Pintsuk f, Michael Rieth a, Steve G. Roberts b, Tobias Weingaertner a a Institute for Applied Materials, Karlsruhe Institute of Technology, Karlsruhe, Germany b Department of Materials, University of Oxford, Oxford, United Kingdom c Department of Materials, Imperial College London, London, United Kingdom d Max-Planck-Institut fuer Plasmaphysik, Garching, Germany e PLANSEE SE, Reutte, Austria f Institute for Energy Research, Forschungszentrum Juelich, Juelich, Germany article info abstract Article history: The physical properties of tungsten such as the high melting point of 3420°C, the high strength and thermal Received 1 April 2015 conductivity, the low thermal expansion and low erosion rate make this material attractive as a plasma facing Revised 23 April 2015 material. However, the manufacturing of such tungsten parts by mechanical machining such as milling and Accepted 30 April 2015 turning is extremely costly and time intensive because this material is very hard and brittle. Powder Injection Molding (PIM) as special process allows the mass production of components, the joining Keywords: of different materials without brazing and the creation of composite and prototype materials, and is an ideal Powder Injection Molding (PIM) tool for scientific investigations. Tungsten This contribution describes the characterization and analyses of prototype materials produced via PIM. -
Aurora Pacific Blob the Great Fire Alaskan Expedition Civsoc Trip to Naples Farewell to Teresa Sergot
I m p e r i a l ENGINEER AURORA PACIFIC BLOB THE GREAT FIRE ALASKAN EXPEDITION CIVSOC TRIP TO NAPLES FAREWELL TO TERESA SERGOT For members of City & Guilds College Association and The Royal School of Mines Association ISSUE 25 AUTUMN 2016 ISSUE TWENTY FIVE Autumn 2016 In this issue… 12 16 28 ASSOCIATION NEWS & REVIEWS FACULTY NEWS 3 Presidents Report 8-9 We bid farewell to Teresa Sergot 4 CGCA AGM Presidential Speech 10 Imperial engineer develops imaging tool as part of 5 Old Centralians’ Trust annual roundup the Human Connectome Project 6-7 FCGI; Back to the Future – reinventing the UK’s 10 Imperial researcher wins Royal Society award manufacturing industry 10 Imperial renews Athena SWAN silver status 7 Our new RSMA President: Tim Cotton 11 Three new Imperial Fellows announced by Royal 30 Book review; Mech. Eng. alumnus brings engineering Academy of Engineering to kids; Tea party in BC; Elec. Eng. 1978-81 class 11 Imperial engineer awarded Young Engineer of the reunion; 1964 City & Guilds Epic Film Year 31 Diary OBITUARIES: FEATURES 31 Prof Bernard G Neal; Pauline Rowe; Timothy Gare 12-14 Tales of an aurora hunter – Melanie Windridge 32 Grosvenor Rex Davis 15 The northern Pacifc Blob – Wyss Yim 33 Karel Labberte; Peter Clark; Clifford Williams 16-20 Alaskan Expedition – Sara Arbós Torrent, Boris Korzh, 34 David Osborne; Peter Ashcroft Sam Thompson, Arnaud Sors 35 Norman Loch; Steve Stephens; Dudley Dennington 21-23 Hydraulic Fracturing in Hydrocarbon Reservoirs – Sharinia Kanagandran Front Cover photo: 23-24 Should a Materials Science -
Annual Review Annex 2018-19
Annual Review Annex 2018/19 Annex to the Annual Review Contents Fellows elected in 2018 . 2 Leaders in Innovation Fellowships . 27 International Fellows . 2 Leaders in Innovation Fellowships Participants . 27 Honorary Fellows . .2 . Global Challenges Research Fund Africa Catalyst . 34. Fellows . .2 . Higher Education Partnerships in sub-Saharan Trustee Board . 4 . Africa . 35 Academy Governance Committees . .5 . Industry-Academia Partnership programme . 37 Academy Operating Committees . 8 UK-China Urban Flooding Research Impact programme . 43 Grants, fellowships and programmes . 11 . Distinguished Visiting Fellowships and Missions Research Chairs . .11 . in Turkey . 44. Research Chairs in Emerging Technologies . 14 Distinguished Visiting Fellowships . .45 . Senior Research Fellowships . 15 . Africa Prize for Engineering Innovation . .46 . Leverhulme Trust Senior Research Fellowships . .16 Frontiers of Development . 47. Daphne Jackson Trust Fellowships . .16 . Ingenious public engagement awards . .48 . Research Fellowships . 17 . Engineering Leaders Scholarships . 49 Engineering for Development Research Visiting Professors . 52. Fellowships . 19. Sainsbury Management Fellowships . 54. UK IC Postdoctorial Research Fellowships . .20 . Connecting STEM Teachers programme . 54 Lloyd’s Register Foundation Research Fellowships . 20. Queen Elizabeth Prize for Engineering . 56 RAEng Industrial Fellowships Scheme . .21 . Panel of judges . 56. APEX Awards . .22 . Foundation Trustees . 57 Enterprise Fellowships . 23 Donors . 57 IRT Enterprise Fellowships . 23 Search group . .58 . Royal Commission 1851 Enterprise Development and fundraising . .59 . Fellowships . 24. Contributors to Academy programmes . 59 . Launchpad Competition . 24 Annual Fund 2018 . 59 SME Leaders programme . 24. Sir Ralph Robins Scholarship Fund . 60 Global Challenges Research Fund Frontiers of Engineering for Development seed funding . 25. 1 Fellows elected in 2018 Fellows of the Academy are leading engineers in the UK drawn from academia, industry and the not-for-profit sectors. -
UNIVERSITY of CALIFORNIA SAN DIEGO Multiscale Characterization
UNIVERSITY OF CALIFORNIA SAN DIEGO Multiscale Characterization Techniques to Elucidate Mechanical Behavior of Materials A dissertation submitted in partial satisfaction of the Requirements for the degree Doctor of Philosophy in Materials Science and Engineering by Chaoyi Zhu Committee in charge Kenneth S. Vecchio, Chair Jian Luo Vlado Lubarda Jeffrey S. Gee Emily Joyce Van Allen 2019 SIGNATURE PAGE The Dissertation of Chaoyi Zhu is approved, and it is acceptable in quality and form for publication and electronically: Chair University of California San Diego 2019 iii DEDICATION In recognition of the guidance and support he has provided over the past four years, this dissertation is dedicated to my advisor, Dr. Kenneth Vecchio. Besides, in recognition of all the love and support they have given me in pursuing my passion, this dissertation is dedicated to my parents Weimin Zhu and Youzhen Xu, and my wife Yidan Hu. iv TABLE OF CONTENTS Contents SIGNATURE PAGE ...................................................................................................................... iii DEDICATION ............................................................................................................................... iv TABLE OF CONTENTS ................................................................................................................ v LIST OF ABBREVIATIONS ....................................................................................................... xii LIST OF FIGURES .................................................................................................................... -
Open Letter to Candidates for General Election
Dear Candidate, To meet the challenge of climate change the United Kingdom needs bold and urgent action. Even as we get smarter in our individual energy use, moving to electric heating, trains and vehicles could double electricity demand by 2050, and all of this needs to be low carbon. We must also protect our quality of life, guaranteeing affordable power for your constituents’ homes, businesses, schools and hospitals. This prompts big questions: • Do we have the means to deliver Net Zero emissions quickly? • Can we make sure our electricity supply is dependable? • Can we create a boom in ‘green jobs’ to support employment as we phase out fossil fuels? The answer is yes, but only if we act now. Renewables are growing, but weather-dependent wind and solar need a low- carbon backbone of stable energy, and only nuclear currently fits the bill. Several smaller reactor designs are developing well and the UK leads the world in the development of fusion. Progress is also being made in battery storage and dealing with waste CO2, but we need to make big decisions now on commercially available, proven technology or we will miss the mark. Modern, large-scale, nuclear power stations are being built around the world today. They will capture their waste, pay for and store it safely and be overseen by national and international regulators and conventions. They can also be used to make hydrogen, a high-efficiency clean fuel that can be used for transportation, heating, and power generation in places where it is difficult to use electricity. More than this, nuclear is an economic powerhouse, employing hundreds of thousands of people directly and in related industries. -
Annual Review Annex 2015-16
Annual Review Annex 2015/2016 Annex to the Annual Review Contents Fellows elected in 2015 2 Engineering Leadership Advanced Awards 27 International Fellows 2 Ingenious Public Engagement Awards 30 Fellows 2 Visiting Professors 31 Sainsbury Management Fellowships 32 Trustee Board 4 Aerospace MSc Bursary Scheme 33 Academy Governance Committees 5 Petrofac Fellowships 34 Academy Operating Committees 8 Panasonic Trust Fellowships 34 Awards 11 Hertha Marks Ayrton Fellowship 34 Sir Angus Paton Bursary 34 Grants, fellowships and programmes 12 Research Chairs 12 Queen Elizabeth Prize for Engineering 35 Research Chairs in Emerging Technologies 14 Panel of judges 35 Senior Research Fellowships 14 Queen Elizabeth Prize for Engineering Foundation Trustees 35 RAEng/EPSRC Research Fellowships 14 Donors 36 Leverhulme Trust Senior Research Fellowships 15 Search group 36 Daphne Jackson Trust Fellowships 15 Development and fundraising 37 RAEng Research Fellowships 16 Development Advisory Board 37 RAEng/Lloyd’s Register Foundation Research Contributors to Academy programmes 37 Fellowship 18 Royal Academy of Engineering RAEng/Ministry of Defence 2015 Annual Fund 38 Research Fellowship 18 Enterprise Fellowships 18 Blavatnik Family Foundation Enterprise Fellowship Alumni Awards 19 Pathways to Growth 19 Launchpad Competition 19 RAEng-ERA Foundation Entrepreneurs Award 20 Distinguished Visiting Fellowships 20 Newton Research Collaboration Programme 22 Leaders in Innovation Fellowships 25 Africa Prize for Engineering Innovation 25 Industrial Secondments Scheme 26 1 Fellows Fellows of the Academy are leading engineers in the UK drawn from academia, industry and the not-for-profit sectors. Fellowship is a national honour, awarded for outstanding personal engineering achievements. Election to the Fellowship is managed by current Fellows of the Academy. -
Nuclear Research and Technology: Breaking the Cycle of Indecision
SCIENCE AND TECHNOLOGY SELECT COMMITTEE Nuclear research and technology: Breaking the cycle of indecision Oral and written evidence Contents AMEC Foster Wheeler (AMEC FW) – Written evidence (PNT0044) ................... 4 Atkins – Written evidence (PNT0015) ....................................................... 14 Atomic Acquisitions (AA) – Written evidence (PNT0040) ............................. 23 Professor Colin Boxall, Lancaster University – Written Evidence (PNT0051) ... 31 Bristol University/SW Nuclear Hub – Written evidence (PNT0043) ................ 37 Professor Grace Burke, University of Manchester, Centre for Nuclear Engineering, Imperial College London and Nuclear Advanced Manufacturing Research Centre (Nuclear AMRC) – Oral evidence (QQ 1-8) ........................ 42 Cambridge Nuclear Energy Centre, University of Cambridge – Written evidence (PNT0056) ............................................................................................ 43 Centre for Nuclear Engineering, Imperial College London – Written evidence (PNT0054) ............................................................................................ 58 Centre for Nuclear Engineering, Imperial College London, Nuclear Advanced Manufacturing Research Centre (Nuclear AMRC) and Professor Grace Burke, University of Manchester – Oral evidence (QQ 1-8) .................................... 66 Dalton Nuclear Institute, University of Manchester – Written evidence (PNT0018) ...........................................................................................