And Large-Scale CO2 Sequestration by Chain-Forming Diatoms in the Sea
Total Page:16
File Type:pdf, Size:1020Kb
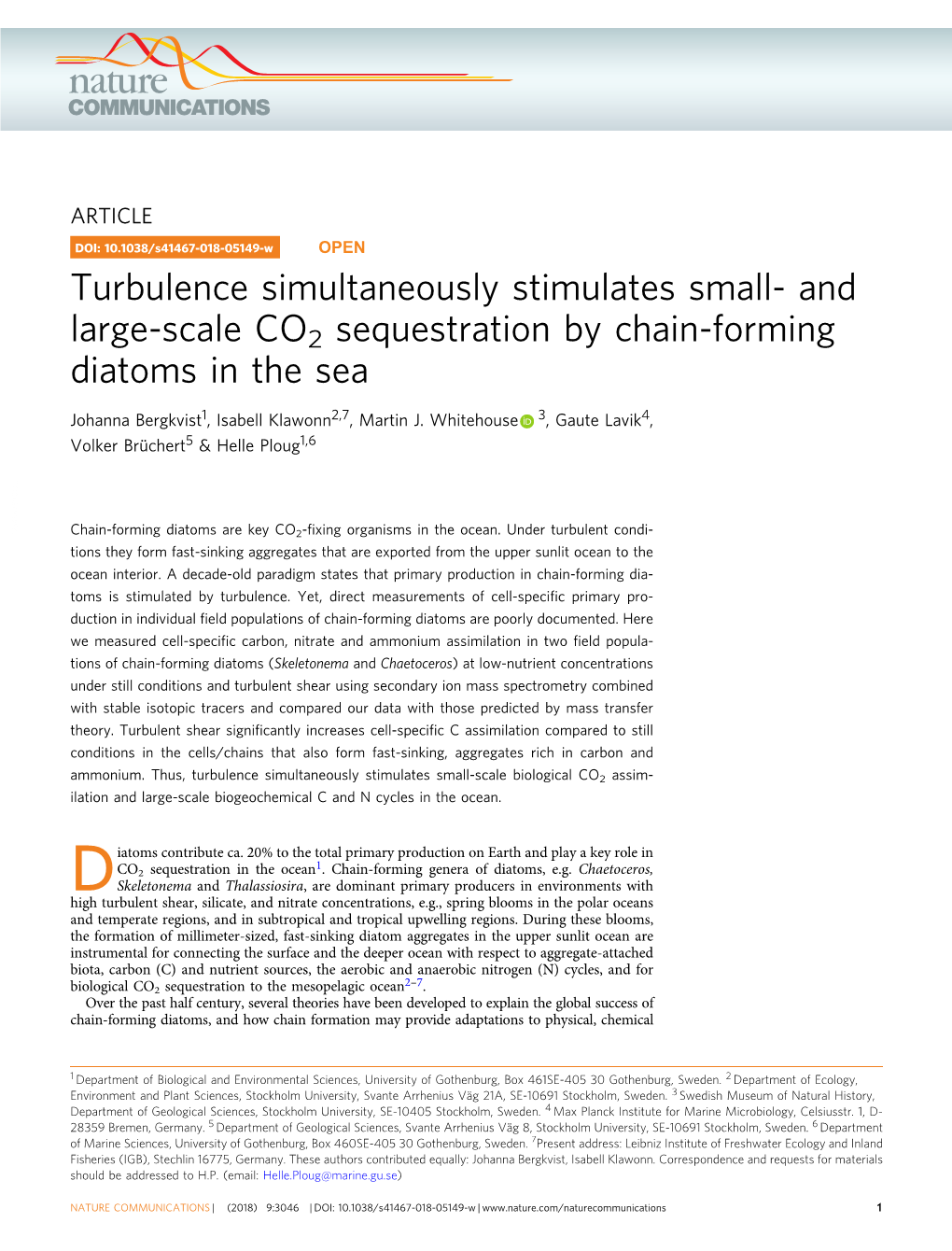
Load more
Recommended publications
-
High Density of Diploria Strigosa Increases
HIGH DENSITY OF DIPLORIA STRIGOSA INCREASES PREVALENCE OF BLACK BAND DISEASE IN CORAL REEFS OF NORTHERN BERMUDA Sarah Carpenter Department of Biology, Clark University, Worcester, MA 01610 ([email protected]) Abstract Black Band Disease (BBD) is one of the most widespread and destructive coral infectious diseases. The disease moves down the infected coral leaving complete coral tissue degradation in its wake. This coral disease is caused by a group of coexisting bacteria; however, the main causative agent is Phormidium corallyticum. The objective of this study was to determine how BBD prominence is affected by the density of D. strigosa, a common reef building coral found along Bermuda coasts. Quadrats were randomly placed on the reefs at Whalebone Bay and Tobacco Bay and then density and percent infection were recorded and calculated. The results from the observations showed a significant, positive correlation between coral density and percent infection by BBD. This provides evidence that BBD is a water borne infection and that transmission can occur at distances up to 1m. Information about BBD is still scant, but in order to prevent future damage, details pertaining to transmission methods and patterns will be necessary. Key Words: Black Band Disease, Diploria strigosa, density Introduction Coral pathogens are a relatively new area of study, with the first reports and descriptions made in the 1970’s. Today, more than thirty coral diseases have been reported, each threatening the resilience of coral communities (Green and Bruckner 2000). The earliest identified infection was characterized by a dark band, which separated the healthy coral from the dead coral. -
Versatile Cyanobacteria Control the Timing and Extent of Sulfide
The ISME Journal (2020) 14:3024–3037 https://doi.org/10.1038/s41396-020-0734-z ARTICLE Versatile cyanobacteria control the timing and extent of sulfide production in a Proterozoic analog microbial mat 1 2,9 1,10 2 3 Judith M. Klatt ● Gonzalo V. Gomez-Saez ● Steffi Meyer ● Petra Pop Ristova ● Pelin Yilmaz ● 4,5,11 6 7 1,8 2 Michael S. Granitsiotis ● Jennifer L. Macalady ● Gaute Lavik ● Lubos Polerecky ● Solveig I. Bühring Received: 28 February 2020 / Revised: 16 July 2020 / Accepted: 28 July 2020 / Published online: 7 August 2020 © The Author(s) 2020. This article is published with open access Abstract Cyanobacterial mats were hotspots of biogeochemical cycling during the Precambrian. However, mechanisms that controlled O2 release by these ecosystems are poorly understood. In an analog to Proterozoic coastal ecosystems, the Frasassi sulfidic springs mats, we studied the regulation of oxygenic and sulfide-driven anoxygenic photosynthesis (OP and AP) in versatile cyanobacteria, and interactions with sulfur reducing bacteria (SRB). Using microsensors and stable isotope probing we found that dissolved organic carbon (DOC) released by OP fuels sulfide production, likely by a specialized SRB population. Increased sulfide fluxes were only stimulated after the cyanobacteria switched from AP to OP. O2 production 1234567890();,: 1234567890();,: triggered migration of large sulfur-oxidizing bacteria from the surface to underneath the cyanobacterial layer. The resultant sulfide shield tempered AP and allowed OP to occur for a longer duration over a diel cycle. The lack of cyanobacterial DOC supply to SRB during AP therefore maximized O2 export. This mechanism is unique to benthic ecosystems because transitions between metabolisms occur on the same time scale as solute transport to functionally distinct layers, with the rearrangement of the system by migration of microorganisms exaggerating the effect. -
Motility of the Giant Sulfur Bacteria Beggiatoa in the Marine Environment
Motility of the giant sulfur bacteria Beggiatoa in the marine environment Dissertation Rita Dunker Oktober 2010 Motility of the giant sulfur bacteria Beggiatoa in the marine environment Dissertation zur Erlangung des Doktorgrades der Naturwissenschaften Dr. rer. nat. von Rita Dunker, Master of Science (MSc) geboren am 22. August 1975 in Köln Fachbereich Biologie/Chemie der Universität Bremen Gutachter: Prof. Dr. Bo Barker Jørgensen Prof. Dr. Ulrich Fischer Datum des Promotionskolloquiums: 15. Dezember 2010 Table of contents Summary 5 Zusammenfassung 7 Chapter 1 General Introduction 9 1.1 Characteristics of Beggiatoa 1.2 Beggiatoa in their environment 1.3 Temperature response in Beggiatoa 1.4 Gliding motility in Beggiatoa 1.5 Chemotactic responses Chapter 2 Results 2.1 Mansucript 1: Temperature regulation of gliding 49 motility in filamentous sulfur bacteria, Beggiatoa spp. 2.2 Mansucript 2: Filamentous sulfur bacteria, Beggiatoa 71 spp. in arctic, marine sediments (Svalbard, 79° N) 2.3. Manuscript 3: Motility patterns of filamentous sulfur 101 bacteria, Beggiatoa spp. 2.4. A new approach to Beggiatoa spp. behavior in an 123 oxygen gradient Chapter 3 Conclusions and Outlook 129 Contribution to manuscripts 137 Danksagung 139 Erklärung 141 Summary Summary This thesis deals with aspects of motility in the marine filamentous sulfur bacteria Beggiatoa and thus aims for a better understanding of Beggiatoa in their environment. Beggiatoa inhabit the microoxic zone in sediments. They oxidize reduced sulfur compounds such as sulfide with oxygen or nitrate. Beggiatoa move by gliding and respond to stimuli like oxygen, light and presumably sulfide. Using these substances for orientation, they can form dense mats on the sediment surface. -
A Model Study of the Effects of Sulfide-Oxidizing Bacteria
View metadata, citation and similar papers at core.ac.uk brought to you by CORE provided by Helsingin yliopiston digitaalinen arkisto Boreal environment research 16: 167–184 © 2011 issn 1239-6095 (print) issn 1797-2469 (online) helsinki 30 June 2011 a model study of the effects of sulfide-oxidizing bacteria (Beggiatoa spp.) on phosphorus retention processes in hypoxic sediments: implications for phosphorus management in the Baltic sea sepehr shakeri Yekta* and lars rahm Department of Thematic Studies — Water and Environmental Studies, Linköping University, SE-581 83 Linköping, Sweden (corresponding author’s e-mail: [email protected]) Received 14 June 2010, accepted 23 Nov. 2010 (Editor in charge of this article: Heikki Seppä) Yekta, s. s. & rahm, l. 2011: a model study of the effects of sulfide-oxidizing bacteria (Beggiatoa spp.) on phosphorus retention processes in hypoxic sediments: implications for phosphorus management in the Baltic sea. Boreal Env. Res. 16: 167–184. Ongoing eutrophication increases phosphorus storage in surficial sediments of the Baltic Sea which can then be released during hypoxic/anoxic events. Such sediments are suit- able habitats for sulfide-oxidizing bacteria,Beggiatoa spp. The objective of this paper is to investigate the effects of these bacteria on the P retention processes in hypoxic sediments using a diagenetic model. This model simulates interactions of the processes controlling P mobility in the sediments with redox reactions from the Beggiatoa metabolism. Modeling results demonstrate that P retention capability is limited when dissolved iron is mineralized as iron sulfides in the sediments. In this regard, sulfide consumption by Beggiatoa spp. potentially decreases the rate of iron sulfide formation and consequently increases the P retention capability in local-scale sediment. -
Trophic Role of Large Benthic Sulfur Bacteria in Mangrove Sediment
Vol. 516: 127–138, 2014 MARINE ECOLOGY PROGRESS SERIES Published December 3 doi: 10.3354/meps11035 Mar Ecol Prog Ser Trophic role of large benthic sulfur bacteria in mangrove sediment Pierre-Yves Pascal1,*, Stanislas Dubois2, Henricus T. S. Boschker3, Olivier Gros1 1Département de Biologie, Université des Antilles et de la Guyane, UMR 7138 UPMC-CNRS-MNHN-IRD, Equipe ‘biologie de la mangrove’, UFR des Sciences Exactes et Naturelles, BP 592, 97159 Pointe-à-Pitre, Guadeloupe, France 2IFREMER, DYNECO Laboratoire d’Ecologie Benthique,29280 Plouzané, France 3Royal Netherlands Institute of Sea Research (NIOZ), PO Box 140, 4400 AC Yerseke, The Netherlands ABSTRACT: Large filamentous sulfur-oxidizing bacteria belonging to the Beggiatoacae family can cover large portions of shallow marine sediments surrounding mangroves in Guadeloupe (French West Indies). In order to assess the importance of Beggiatoa mats as an infaunal food source, observations were conducted of the area within mats and at increasing distances from mats. We used natural isotopic compositions and a 13C enrichment study. Both revealed an inges- tion of bacterial mats by associated meiofauna, dominated by rotifers and to a smaller extent by small polychaetes and nematodes. Compared to adjacent sites, sediment covered by bacterial mats presented a higher abundance of diatoms, whereas the total biomass of bacteria did not vary. This constant bacterial abundance suggests that the proportion of organic matter represented by sulfur bacteria is limited compared to the fraction of total bacteria. There was no significant differ- ence in infaunal abundance in mats, suggesting that the availability of this chemosynthetic food resource had a limited local effect. -
Insights Into Marine Microbial Communities That Couple Anaerobic
Goldschmidt Conference Abstracts 919 Insights into marine microbial Aerobic hydrogen oxidation by a communities that couple anaerobic chemolithotrophic Beggiatoa strain biogeochemical cycles to remote A.-C. GIRNTH* AND H.N. SCHULZ-VOGT oxidants Max Planck Institut for Marine Microbiology, Celsiusstraße 1, 1 2 28357 Bremen, Germany PETER R. GIRGUIS *, PENGFEI SONG AND 2 (*correspondence: [email protected]) MARK NIELSEN 1Harvard University, 16 Divinity Avenue Room 3085, Hydrogen oxidation in oxic/anoxic gradients Cambridge, MA 02138 (* correspondence: Transition zones between oxic and anoxic environments [email protected]) are primary habitats for aerobic hydrogen oxidizers. In sulfidic 2Harvard University, 16 Divinity Avenue Room 3092, sediments, filamentous bacteria of the genus Beggiatoa thrive Cambridge, MA 02138 within this narrow horizon and feature fine-tuned chemotactic responses to keep track of the interface. So far, only anaerobic hydrogen oxidation coupled to reduction of stored sulfur has Extracellular electron transfer (EET) is a process whereby been shown for a heterotrophic Beggiatoa strain [1]. Here we microbes shuttle electrons outside the cell, and access solid- demonstrate aerobic hydrogen oxidation by the phase oxidants as well as spatially remote oxidants. EET has chemolithoautotrophic strain Beggiatoa 35Flor grown in a been well-studied in cultivated microbes, e.g., heterotrophic mineral medium featuring artificial oxygen, sulfide and iron-reducing "-proteobacteria. The relevance of EET in hydrogen gradients. nature, however, and its impact on biogeochemical cycles remains poorly constrained. Anaerobic marine sediments host Beggiatoa 35Flor uses hydrogen as an accessory microbial communities that are involved in numerous electron donor biogeochemical cycles, and those capable of EET would have In the presence of hydrogen, Beggiatoa 35Flor mats access to solid-phase as well as remote oxidants. -
The Biology of Beggiatoa
Louisiana State University LSU Digital Commons LSU Historical Dissertations and Theses Graduate School 1980 The iologB y of Beggiatoa. William Roy Strohl Louisiana State University and Agricultural & Mechanical College Follow this and additional works at: https://digitalcommons.lsu.edu/gradschool_disstheses Recommended Citation Strohl, William Roy, "The ioB logy of Beggiatoa." (1980). LSU Historical Dissertations and Theses. 3544. https://digitalcommons.lsu.edu/gradschool_disstheses/3544 This Dissertation is brought to you for free and open access by the Graduate School at LSU Digital Commons. It has been accepted for inclusion in LSU Historical Dissertations and Theses by an authorized administrator of LSU Digital Commons. For more information, please contact [email protected]. INFORMATION TO USERS This was produced from a copy of a document sent to us for microfilming. While the most advanced technological means to photograph and reproduce this document have been used, the quality is heavily dependent upon the quality of the material submitted. The following explanation of techniques is provided to help you understand markings or notations which may appear on this reproduction. 1. The sign or “target” for pages apparently lacking from the document photographed is “Missing Page(s)” . If it was possible to obtain the missing page(s) or section, they are spliced into the film along with adjacent pages. This may have necessitated cutting through an image and duplicating adjacent pages to assure you of complete continuity. 2. When an image on the film is obliterated with a round black mark it is an indication that the film inspector noticed either blurred copy because of movement during exposure, or duplicate copy. -
Fine-Structural Analysis of Black Band Disease-Infected Coral Reveals
Vol. 93: 179–190, 2011 DISEASES OF AQUATIC ORGANISMS Published February 22 doi: 10.3354/dao02305 Dis Aquat Org Fine-structural analysis of black band disease- infected coral reveals boring cyanobacteria and novel bacteria Aaron W. Miller1,*, Patricia Blackwelder2, 3, 4, Husain Al-Sayegh2, 3, Laurie L. Richardson1 1Department of Biological Sciences, Florida International University, Miami, Florida 33199, USA 2University of Miami Center for Advanced Microscopy (UMCAM), Miami, Florida 33124, USA 3Marine Geology and Geophysics, University of Miami, Miami, Florida 33149, USA 4Nova Southeastern University Oceanographic Center, Dania, Florida 33004, USA ABSTRACT: Examination of coral fragments infected with black band disease (BBD) at the fine- and ultrastructural levels using scanning (SEM) and transmission electron microscopy (TEM) revealed novel features of the disease. SEM images of the skeleton from the host coral investigated (Montas- traea annularis species complex) revealed extensive boring underneath the BBD mat, with cyanobac- terial filaments present within some of the bore holes. Cyanobacteria were observed to penetrate into the overlying coral tissue from within the skeleton and were present throughout the mesoglea between tissue layers (coral epidermis and gastrodermis). A population of novel, as yet unidentified, small filamentous bacteria was found at the leading edge of the migrating band. This population increased in number within the band and was present within degrading coral epithelium, suggesting a role in disease etiology. In coral tissue in front of the leading edge of the band, cyanobacterial filaments were observed to be emerging from bundles of sloughed-off epidermal tissue. Degraded gastrodermis that contained actively dividing zooxanthellae was observed using both TEM and SEM. -
Polyphosphate in Marine Environments and Beggiatoa Sp
Polyphosphate in Marine Environments and Beggiatoa sp. – Quantification and Visualization Dissertation zur Erlangung des akademischen Grades Doctor rerum naturalium (Dr. rer. nat.) der Mathematisch-Naturwissenschaftlichen Fakultät der Universität Rostock vorgelegt von Simon Langer Rostock, August 2019 Tag der Einreichung: 23.08.2019 Tag der Verteidigung: 01.11.2019 1. GutachterIn: Prof. Dr. Heide Schulz-Vogt Leibniz-Institut für Ostseeforschung Warnemünde Geomikrobiologie 2. GutachterIn: PD Dr. Stefan Forster Universität Rostock Meeresbiologie Table of content Zusammenfassung III Summary 1 1 Introduction 3 1.1 Microbial phosphorus cycling ......................................................................................... 3 1.2 Polyphosphate ................................................................................................................. 4 1.3 Polyphosphate habitats .................................................................................................... 6 1.4 Challenges in (poly)P research ........................................................................................ 7 1.5 Aims of this thesis ........................................................................................................... 9 2 Material and Methods 11 2.1 Study areas .................................................................................................................... 11 2.1.1 Hütelmoor and Baltic Sea ................................................................................. 11 2.1.2 Upwelling and -
Bioglea As a Source of Bioactive Ingredients: Chemical and Biological Evaluation
cosmetics Article Bioglea as a Source of Bioactive Ingredients: Chemical and Biological Evaluation Marisanna Centini 1,*, Mario Roberto Tredici 2, Natascia Biondi 2, Anna Buonocore 1, Roberto Maffei Facino 3 and Cecilia Anselmi 1 1 Dipartimento di Biotecnologie, Chimica e Farmacia, University of Siena, Via Aldo Moro 2, 53100 Siena, Italy; [email protected] (A.B.); [email protected] (C.A.) 2 Dipartimento di Scienze e Tecnologie Agrarie, Alimentari, Ambientali e Forestali (DAGRI), University of Florence, Piazzale delle Cascine 24, 50144 Florence, Italy; mario.tredeci@unifi.it (M.R.T.); natascia.biondi@unifi.it (N.B.) 3 Dipartimento di Scienze Farmaceutiche “Pietro Pratesi”, University of Milan, Via Mangiagalli 25, 20133 Milan, Italy; roberto.maff[email protected] * Correspondence: [email protected] Received: 3 September 2020; Accepted: 23 October 2020; Published: 27 October 2020 Abstract: This study focused on bioglea in thermal material sampled at Saturnia spa (Tuscany, Italy). Bioglea is the term used to define the thermal plankton consisting of biogenic substances that have been investigated little from the chemical and biological points of view. Bioglea is mainly formed of cyanobacteria, particularly from the Oscillatoriales subsection, and it seems to have an important role in the maturation of thermal mud for the development of organic matter. This cyanobacteria-dominated community develops in a large outdoor pool at the spa, where the spring water is collected, over the sediments, with matter floating at the surface. Throughout the year, the cyanobacterial species of bioglea were the same, but their relative abundance changed significantly. For chemical characterization an extractive method and several analytical techniques (HPLC, GC-MS, SPME) were used. -
Characterization of Oscillatoria Spp. and Their Role in Black Band Disease of Coral Dina Stanic Florida International University, [email protected]
Florida International University FIU Digital Commons FIU Electronic Theses and Dissertations University Graduate School 7-12-2010 Characterization of Oscillatoria spp. and their Role in Black Band Disease of Coral Dina Stanic Florida International University, [email protected] DOI: 10.25148/etd.FI10080901 Follow this and additional works at: https://digitalcommons.fiu.edu/etd Part of the Life Sciences Commons Recommended Citation Stanic, Dina, "Characterization of Oscillatoria spp. and their Role in Black Band Disease of Coral" (2010). FIU Electronic Theses and Dissertations. 243. https://digitalcommons.fiu.edu/etd/243 This work is brought to you for free and open access by the University Graduate School at FIU Digital Commons. It has been accepted for inclusion in FIU Electronic Theses and Dissertations by an authorized administrator of FIU Digital Commons. For more information, please contact [email protected]. FLORIDA INTERNATIONAL UNIVERSITY Miami, Florida CHARACTERIZATION OF OSCILLATORIA SPP. AND THEIR ROLE IN BLACK BAND DISEASE OF CORAL A thesis submitted in partial fulfillment of the requirements for the degree of MASTER OF SCIENCE in BIOLOGY by Dina Stanic 2010 To: Dean Kenneth Furton College of Arts and Sciences This thesis, written by Dina Stanic, and entitled Characterization of Oscillatoria spp. and their Role in Black Band Disease of Coral, having been approved in respect to style and intellectual content, is referred to you for judgment. We have read this thesis and recommend that it be approved. _______________________________________ Miroslav Gantar _______________________________________ John Makemson _______________________________________ Laurie L. Richardson, Major Professor Date of Defense: July 12, 2010 The thesis of Dina Stanic is approved. -
Sulfur-Cycling Fossil Bacteria from the 1.8-Ga Duck Creek Formation Provide Promising Evidence of Evolution’S Null Hypothesis
Sulfur-cycling fossil bacteria from the 1.8-Ga Duck Creek Formation provide promising evidence of evolution’s null hypothesis J. William Schopfa,b,c,d,e,1, Anatoliy B. Kudryavtsevb,d,e, Malcolm R. Walterf,g, Martin J. Van Kranendonkf,h,i, Kenneth H. Williforde,j,k, Reinhard Kozdone,j, John W. Valleye,j, Victor A. Gallardol, Carola Espinozal, and David T. Flanneryf,g,k aDepartment of Earth, Planetary, and Space Sciences, bCenter for the Study of Evolution and the Origin of Life, and cMolecular Biology Institute, University of California, Los Angeles, CA 90095; dPenn State Astrobiology Research Center, University Park, PA 16802; eAstrobiology Research Consortium and jDepartment of Geoscience, University of Wisconsin−Madison, Madison, WI 53706; fAustralian Centre for Astrobiology, gSchool of Biotechnology and Biomolecular Sciences, hSchool of Biological, Earth and Environmental Sciences, and iAustralian Research Council Centre of Excellence for Core to Crust Fluid Systems, University of New South Wales, Randwick, NSW 2052, Australia; kJet Propulsion Laboratory, California Institute of Technology, Pasadena, CA 91109; and lDepartamento de Oceanografía, Facultad de Ciencias Naturales y Oceanográficas, Universidad de Concepción, Concepción, Chile Edited by Thomas N. Taylor, University of Kansas, Lawrence, KS, and approved January 5, 2015 (received for review October 6, 2014) The recent discovery of a deep-water sulfur-cycling microbial biota from the ∼2.4- to ∼2.2-Ga Great Oxidation Event, the “GOE” in the ∼2.3-Ga Western Australian Turee Creek Group opened a (5). Further, the Duck Creek fossils evidence the “hypo- new window to life’s early history. We now report a second such bradytely” of early-evolved microbes, an exceptionally low rate of subseafloor-inhabiting community from the Western Australian discernable evolutionary change and a term coined (6) to parallel ∼1.8-Ga Duck Creek Formation.