Ultrasound Microbubble Contrast Agents: Fundamentals and Application to Gene and Drug Delivery
Total Page:16
File Type:pdf, Size:1020Kb
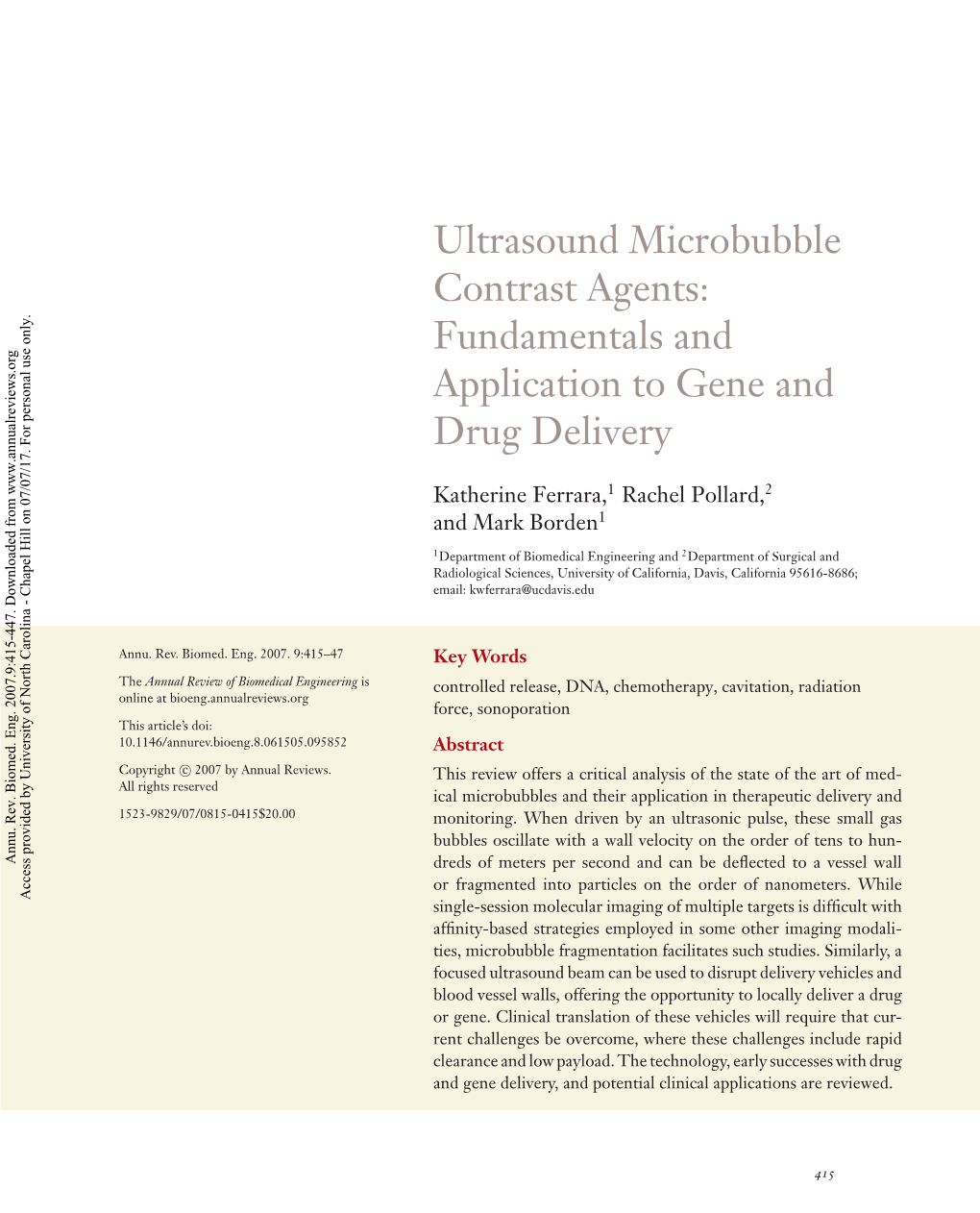
Load more
Recommended publications
-
Assessing the Barriers to Imageguided Drug Delivery
Opinion Assessing the barriers to image-guided drug delivery Gregory M. Lanza,1 Chrit Moonen,2 James R. Baker, Jr.3 Esther Chang,4 Zheng Cheng,5 Piotr Grodzinski,6 Katherine Ferrara,7 Kullervo Hynynen,8 Gary Kelloff,6 Yong-Eun Koo Lee,3 Anil K. Patri,9 David Sept,3 Jan E. Schnitzer,10 Bradford J. Wood,11 Miqin Zhang,12 Gang Zheng13 and Keyvan Farahani6∗ Imaging has become a cornerstone for medical diagnosis and the guidance of patient management. A new field called image-guided drug delivery (IGDD) now combines the vast potential of the radiological sciences with the delivery of treatment and promises to fulfill the vision of personalized medicine. Whether imaging is used to deliver focused energy to drug-laden particles for enhanced, local drug release around tumors, or it is invoked in the context of nanoparticle- based agents to quantify distinctive biomarkers that could risk stratify patients for improved targeted drug delivery efficiency, the overarching goal of IGDD is to use imaging to maximize effective therapy in diseased tissues and to minimize systemic drug exposure in order to reduce toxicities. Over the last several years, innumerable reports and reviews covering the gamut of IGDD technologies have been published, but inadequate attention has been directed toward identifying and addressing the barriers limiting clinical translation. In this consensus opinion, the opportunities and challenges impacting the clinical realization of IGDD-based personalized medicine were discussed as a panel and recommendations were proffered to accelerate the field forward. © 2013 Wiley Periodicals, Inc. How to cite this article: WIREs Nanomed Nanobiotechnol 2014, 6:1–14. -
2020 VASCULAR RESEARCH INITIATIVES CONFERENCE VRIC Chicago 2020: from Discovery to Translation
CANCELED 2020 VASCULAR RESEARCH INITIATIVES CONFERENCE VRIC Chicago 2020: From Discovery to Translation MONDAY, MAY 4, 2020 7:00 AM REGISTRATIO N AND CONTINENTAL BR EAKFAST 8:00 AM INTRODUCTORY REMARKS Luke Brewster, MD, Chair, Research and Education Committee Ali AbuRahma, MD, SVS Vice-President Peter Lawrence, MD, SVS Foundation Alan Dardik, MD, PhD, Editor, JVS-Vascular Science ABSTRACT SESSION I: ARTERIAL REMODELING AND DISCOVERY SCIENCE FOR VENOUS DISEASE Moderator: Ulka Sachdev, MD Moderator: Sanjay Misra, MD 8:15 AM ^* Elastic Fibers Of The Internal Elastic Lamina Are Unraveled But Not Created With Expanding Arterial Diameter In Arteriogenesis Derek Afflu, Univ of Pittsburgh Medical Ctr, PITTSBURGH, PA; Dylan D McCreary, Nolan Skirtich, VA PHS, Pittsburgh, PA; Kathy Gonzalez, UPMC, Pittsburgh, PA; Edith Tzeng, UNIVERSITY PITTSBURGH, Pittsburgh, PA; Ryan M McEnaney VA PHS, Pittsburgh, PA 8:25 AM Loss of Myeloid Specific Protein Phosphatase 2a Accelerates Experimental Venous Thrombus Resolution Andrea T Obi, Renee Beardslee, Catherine Luke, UNIVERSITY OF MICHIGAN, Ann Arbor, MI; Andrew Kimball, Univ of Alabama Birmingham, Birmingham, AL; Abigail R Dowling, Qing Cai, Sriganesh Sharma, UNIVERSITY OF MICHIGAN, Ann Arbor, MI; Katherine A Gallagher, Peter Henke, UNIVERSITY MICHIGAN, Ann Arbor, MI; Bethany Moore 8:35 AM A Synthetic Resolvin Analogue (Benzo-Rvd1) Attenuates Vascular Smooth Muscle Cell (VSMC) Migration And Neointimal Hyperplasia Evan C Werlin, Alexander Kim, SMITH CARDIOVASCULAR RESEARCH BLDG, San Francisco, CA; Hideo Kagaya, -
Radiology Department Report 2017–2019
Radiology Department Report 2017–2019 Radiology Department Report 2017–2019 Contents Letter from the Chair 2 Clinical Divisions 84 In Memoriam 6 Research Divisions 92 Canary Center at Stanford for Cancer Radiology Faculty 8 Early Detection 94 Department Leadership 10 Integrative Biomedical Imaging All Faculty 14 Informatics at Stanford (IBIIS) 100 New Faculty Appointments 18 Molecular Imaging Program at Stanford (MIPS) 106 Faculty Leadership Appointments 26 Precision Health and Integrated New Chair of Biomedical Data Science 27 Diagnostics (PHIND) Center at Stanford 120 radiology.stanford.edu Faculty Retirements and Recalls 28 Radiological Sciences Laboratory (RSL) 128 Faculty Departures 29 Faculty Honors and Awards 30 Feature: IMMERS 33 Active Sponsored Research 138 Future Faculty and Staff 34 Funded Projects Summary 149 Feature: 3DQ Lab 37 Equipment 38 Radiology Snapshot 150 Feature: Industry Collaborations 40 Radiology Family 152 Translational Research 42 Artificial Intelligence in Radiology 44 Thank You to our Colleagues 154 Theragnostics: Combining Diagnostic and Therapeutic Radiopharmaceuticals to Fight Cancer 50 Acknowledging our Diagnostic Ultrasound through a Different Lens 52 Generous Supporters 156 Cover Image Training Programs 54 Shear waves were induced in a cylindrical gelatin phantom using a mechanical vibra- Clinical Training Programs 56 How you can tor, causing them to scatter and reflect. Their displacements were imaged using a phase- Graduating Residents 60 Support the Department 157 contrast 3T MRI technique (MR elastography). This work highlights the importance of image reconstruction algorithms. Each individual wave image (represented by each Graduating Fellows 64 circle) is chaotic and difficult to interpret by itself. However, thousands of wave images can Research Training Programs 69 Canary Challenge 158 be thoughtfully fused together using an image reconstruction algorithm to produce a single Graduating PhDs 73 image representing the gelatin’s mechanical properties. -
Focused Ultrasound Foundation Symposium Summary 2016 (PDF)
Focused Ultrasound Foundation Contents 2 Welcome Thursday, September 1, 2016 2 Honorary Presentations 35 The Journal of Therapeutic Ultrasound 35 Panel Discussion: Biomechanisms 3 Keynote Speakers and Special Guests 36 Collaboration and Data Sharing . 36 Panel Discussion: Successful FUS Sites Monday, August, 29, 2016 37 Regulatory Science 4 Brain 38 Panel Discussion: Evidence Building 4 Movement Disorders – Clinical . 6 Panel Discussion: Movement Disorders 7 Parkinson’s Disease – Preclinical 39 Closing Remarks 7 Alzheimer’s Disease . 8 Psychosurgery 9 Epilepsy 40 Awards 9 Blood-Brain Barrier Opening 40 Ferenc Jolesz Memorial Award 10 Brain – Technical 41 Visionary Award 12 Panel Discussion: Brain Technology 13 Neuromodulation 42 Young Investigator Awards Program 14 Brain Summary 46 FUS Foundation Internship Programs 46 Summer Internship Program Tuesday, August 30, 2016 47 2015 Global Interns 16 Cancer 48 2016 Global Interns 16 Brain Tumors 18 Immunotherapy . 19 Panel Discussion: Immunotherapy 49 Sponsors 20 Liver/Pancreas – Technical 49 Acknowledgements 21 Liver/Pancreas – Clinical 50 Platinum Sponsors 22 Prostate 51 Silver Sponsors 24 Bone Tumors 52 Exhibitors and Supporters 55 Partners Wednesday, August, 31, 2016 27 Breast Tumors 57 Advertisements 27 Other Tumors 28 Drug Delivery for Cancer 30 Cardiovascular Video presentations 31 Women’s Health/Gynecological To view the presentations, click on the bolded 32 Musculoskeletal presenter names. 33 Emerging Applications 34 Technical Topics Focused Ultrasound 5th International Symposium 1 Focused Ultrasound Foundation Welcome Neal F. Kassell, MD Kassell welcomed the over 400 participants to the Symposium, which coincides with the Focused Founder and Chairman, Ultrasound Foundation’s 10th anniversary. In the past 10 years, the growth in the field has Focused Ultrasound Foundation been astounding. -
RISE Executive Summaries Oct 2013
UC Davis Grand Challenges Executive Summaries Oct. 2013- Sept. 2014 Research Investments in the Sciences & Engineering (RISE) Structural Biochemistry of Plant-Pathogen Interactions to Promote Healthy Crops and Enhance Global Food Security Team lead: George Bruening Co-leads: Gitta Coaker, S.P. Dinesh-Kumar, Andrew Fisher, Ioannis Stergiopoulos, David Wilson Team vision: In this banner year of agricultural production, it is easy to miss the longer term trend: world grain production increases have fallen behind world population increases since 1990. Rising middle and upper-classes worldwide are increasing the demand for animal and other high quality foods. Only a small fraction of microbes are plant pathogens, and only specific pairs of plant and plant pathogenic microbe result in an infection. Mechanistic understanding, at the atomic level, of how this interaction occurs is the core goal of this RISE theme. This understanding is expected to create the power to redirect effector-immune receptor interactions or to enhance or diminish their strength, with the practical goal of enhancing crop protection. Goals: Mechanistic understanding, at the atomic level, of how this interaction occurs between pathogen molecules and the plant molecules that recognize them is the core goal of this RISE theme. This understanding is expected to create the power to redirect effector-immune receptor interactions or to enhance or diminish their strength, with the practical goal of enhancing crop protection. The goals as stated in our original proposal are: Determine the crystal structures of several immune complex components singly and as biologically relevant complexes. Determine the biological activities of immune complex components and the intra- and inter-molecular changes induced during immune receptor activation. -
Mark Andrew Borden, Ph.D
Mark Andrew Borden 1111 Engineering Drive, Boulder, CO 80309-0427 Tel/ 303.492.7750; Email/ [email protected] Education 2003 Ph.D. in Chemical Engineering, University of California, Davis 1999 B.S. in Chemical Engineering, University of Arizona, Tucson Experience 2013 – present Associate Professor Department of Mechanical Engineering, University of Colorado, Boulder 2012 – present Fellow Materials Science and Engineering Program, University of Colorado, Boulder 2011 – present Affiliate Faculty Bioengineering, University of Colorado, Denver 2010-2013 Assistant Professor Department of Mechanical Engineering, University of Colorado, Boulder 2007-2010 Assistant Professor Department of Chemical Engineering, Columbia University 2005-2007 Visiting Scientist Department of Radiology, University of Arizona Advisor: Robert Gillies, Ph.D. 2003-2007 Project Scientist Department of Biomedical Engineering, UC Davis Advisor: Katherine Ferrara, Ph.D. 1999-2003 Graduate Research Assistant Department of Chemical Engineering, UC Davis Advisor: Marjorie Longo, Ph.D. 1998-1999 Undergraduate Research Assistant Department of Electrical Engineering, University of Washington Advisor: Deirdre Meldrum, Ph.D. 1997-1998 Undergraduate Research Assistant Department of Chemical Engineering, University of Arizona Advisor: Roberto Guzman, Ph.D. Honors & Awards 2002, 2003 Exxon Summer Graduate Research Fellowship 2004 Professors for the Future Fellowship 2006 Travel Award & Plenary Session, Society for Molecular Imaging 2008 James D. Watson Investigator Award 2010 -
Management Plan Description and Personnel UC
UC Davis ADVANCE: Institutional Transformation to Build and Sustain a Diverse Community of Innovative STEM Scholars Management Plan Description and Personnel Diana Bilimoria Refugio Rochin UC Davis ADVANCE EXTERNAL Carlos Castillo-Chavez Abigail Stewart PROGRAM LEADER ADVISORY Olivia Graeve Caroline S. T. Turner Principal Investigator Brian Nosek Ruth Zambrana BOARD Chancellor Linda Katehi Mariko Chang EXTERNAL EVALUATOR STEERING COMMITTEE Chair: Enrique Lavernia, COE Dean VP-AA Maureen Stanton (Co-PI), Co- Helene Dillard, CAES Dean Chair INTERNAL James E. K. Hildreth, CBS Dean ADVISORY Raymond Rodriguez (Co-PI) Alexandra Navrotsky, MPS Dean BOARD Faculty Director: George R. Mangun, DSS Dean Karen McDonald Rahim Reed, Associate Executive Vice Chancellor Bruno Nachtergaele, Chair Davis Division Academic Senate Associate Director: Sheryl Soucy-Lubell, Director, Interdisciplinary Research Services Kim Shauman (Co-PI) Terry Westover, Director, Center for Education Initiative Directors: & Evaluation Services INTERNAL Linda Bisson Lisa Sullivan, Evaluation Analyst, Center for EVALUATORS Adela de la Torre (Co-PI) Education & Evaluation Services Mary Lou de Leon Siantz Jonathan Eisen Denneal Jamison-McClung, Program Coordinator JoAnne Engebrecht Sophie Barbu, Program Assistant MANAGEMENT Carol Erickson Manju Kaul, Budget Analyst, COE TEAM Susan Rivera Linda Zhao, Financial Analyst, COE Pia Donaldson, Purchasing & Accounts Payable, COE Build a STEM Empower Establish an Understand Barriers Research Career Inclusive & Equitable & Catalysts for Community -
COMPUTING RESEARCH NEWS a Publication of the Computing Research Association
COMPUTING RESEARCH NEWS A Publication of the Computing Research Association January 2003 Vol. 15/No. 1 NIBIB at NIH Promotes Collaboration in Research By Donna J. Dean Overview As the Institute continues to Biomedical Implant Science (BMIS) expand and prosper, areas of Coordinating Committee. The establishment of the National considerable interest include The development of a new Institute of Biomedical Imaging and biomaterials, nanoscience, platform generation of research scientists is Bioengineering (NIBIB) clearly is development, surgery, bioinformatics, also critical to the successful future of having a positive impact on the other multimodality imaging, imaging trans-disciplinary research. Therefore, institutes and centers that comprise devices and agents, computer model- the NIBIB has taken a lead role in the National Institutes of Health. As ing, image-guided therapies and the development of methods for it enters its third year, the NIBIB’s interventions, imaging reconstruc- training researchers who are techni- unique status as an institute dedi- tion, and the physics and mathemat- cally competent in the field, inde- Donna J. Dean cated to emerging biomedical tech- ics of biomedical imaging. Areas of pendent thinkers, successful will be paramount to fully under- nologies is leading to innovative and interest in the technology arena communicators, team players, and standing the mechanisms of all eye collaborative science that crosscuts include sensors, nanotechnology, visionaries in transcending discipli- diseases and disorders. -
Jnm Fm a MIC 22..22
MOLECULAR IMAGING UPDATE NEWSLINE Ultrasound Molecular Imaging: On the Move eports of new agents and systems for ultrasound A second imaging mode, also molecular imaging are rapidly increasing. Stabilized proposed for fast ultrasound molec- Rgas bubbles (;1 micron in diameter) are frequently ular imaging by combining high and used as ultrasound contrast agents, typically remaining low frequency ultrasound pulses, will within blood vessels and targeting receptors on the vessel be reported in ‘‘Radial Modulation wall (1–6). Photoacoustic technologies show potential to Imaging of Microbubbles at High broaden the targets for molecular imaging with acoustic Frequency’’ by Emmanuel Cherin, waves, but are not yet widely available (7). Integration of representing the laboratory of Stuart molecular microbubble imaging into preclinical scientific Foster, at the University of Toronto Katherine Ferrara, PhD studies has been advanced recently by the platform agent for (Ontario,Canada).Specificmicrobub- preclinical imaging commercially available from Targeson, ble targets, including integrins and LLC (Charlottesville, VA). Targeson has also recently an- selectins, will be reported by Kwon-Ha Yoon and Muzaffer nounced the completion of a licensing arrangement with Celebi, respectively. SibTech, Inc. (Newington, CT), facilitating a new line of Two examples of research in the new areas of targeted preclinical microbubble products targeted to VEGFR-2. photoacoustic and thermoacoustic imaging include those of Commercial ultrasound systems also continue to -
Focused Ultrasound Foundation Symposium 2020 Proceedings
7th InternationalFocused SymposiumUltrasound on FocusedPsychiatric Ultrasound Working Group Meeting November 9–13, 2020 Virtual Meeting 18–20 October 2017 Vaughan Estates Sunnybrook Health Sciences Centre Toronto, Canada Westin Prince Hotel Toronto, Canada Sponsored by Focused Ultrasound Foundation vi 7th International Symposium on Focused Ultrasound | Virtual Meeting November 9–13, 2020 Focused Ultrasound Foundation Contents Monday, November 9, 2020 4 Welcome 5 Honorary President’s Address 6 Keynote Speaker Ralph Northam, Governor of Virginia 7 Movement Disorders 7 Oral Presentation Q&A 9 Parkinson’s Disease 9 Panel 12 Neurodegenerative Diseases 12 Special Lecture 13 Oral Presentation Q&A 16 Panel 18 Epilepsy 18 Oral Presentation Q&A 19 Panel 21 Psychiatric Disorders 21 Special Lecture 22 Oral Presentation Q&A 24 Panel 27 Neuromodulation 27 Oral Presentation Q&A 28 Panel Focused Ultrasound for Neuromodulation Considerations for Human Translation Panel . Tuesday, November 10, 2020 33 Brain Tumors 33 Oral Presentation Q&A 36 Panel 39 Keynote Speaker James Allison, MD, Anderson Cancer Center 41 Cancer Immunotherapy 41 Brain Oral Presentation Q&A 43 Breast Oral Presentation Q&A 46 Other Oral Presentation Q&A 49 Panel 52 Fireside Chat Cancer Therapy: Beyond Ionizing Radiation 53 Special Lecture Liquid Biopsy 54 Special Session International Society for Theraputic Ultrasound (ITSU) . 7th International Symposium on Focused Ultrasound | Virtual Meeting November 9–13, 2020 1 Focused Ultrasound Foundation Wednesday, November 11, 2020 56 Keynote Speaker Michael Milken, Milken Institute 56 Breast Cancer 56 Panel 58 Prostate 58 Special Lecture 59 Oral Presentation Q&A 61 Panel 65 2020 Awards Ceremony 65 Visionary Award 66 Ferenc Jolesz Memorial Award 67 Andrew J. -
Table of Contents (PDF)
1416 Diagnosis of Diffuse and Localized Arrhythmogenic Right Ventricular Dysplasia by Gated Blood-Pool SPECT Denis Mariano-Goulart, Laurent Dechaux,´ Francxois Rouzet, Eric Barbotte, Charles Caderas de Kerleau, Michel Rossi, and Dominique Le Guludec Volume 48, Number 9 • September 2007 1424 Added Value of Coronary Artery Calcium Score as an Adjunct to Gated SPECT for the Evaluation of Coronary SNM NEWSLINE Artery Disease in an Intermediate-Risk Population Tiziano Schepis, Oliver Gaemperli, Pascal Koepfli, Mehdi Namdar, 13N Commentary: Are You Ready? Ines Valenta, Hans Scheffel, Sebastian Leschka, Lars Husmann, Robert E. Henkin Franz R. Eberli, Thomas F. Luscher, Hatem Alkadhi, and Philipp A. Kaufmann 14N SNM Salutes Outstanding Contributors at 54th Annual Meeting 1431 Application of 99mTc-Pertechnetate Scintigraphy to Microvascular Autologous Transplantation of the 18N Watson Receives Loevinger–Berman Award Submandibular Gland in Patients with Severe George Sgouros Keratoconjunctivitis Sicca Lei Zhang, Zheng-Hong Zhu, Hao-Jie Dai, Zhi-Gang Cai, Chi Mao, Xin Peng, and Guang-Yan Yu 22N Molecular Imaging Update: Ultrasound Molecular Imaging: On the Move 1436 Tumor Imaging Using 1-(29-deoxy-29-18F-Fluoro-b-D- Katherine Ferrara Arabinofuranosyl)Thymine and PET Omid S. Tehrani, Otto Muzik, Lance K. Heilbrun, Kirk A. Douglas, 27N Maintenance of Certification Update: Part IV of MOC: Jawana M. Lawhorn-Crews, Haihao Sun, Thomas J. Mangner, What Is It? and Anthony F. Shields Henry D. Royal 1442 Role of 99mTc-Octreotide Acetate Scintigraphy in 28N SNM Leadership Update: How Do We Introduce the Suspected Lung Cancer Compared with 18F-FDG Next Generation of Radiotracers into Clinical Practice? Dual-Head Coincidence Imaging Alexander J. -
Molecular Imaging in Nanotechnology and Theranostics Chrysafis Andreou,1 Suchetan Pal,1 Lara Rotter,2 Jiang Yang,1 Moritz F
Mol Imaging Biol (2017) 19:363Y372 DOI: 10.1007/s11307-017-1056-z * World Molecular Imaging Society, 2017 Published Online: 27 March 2017 SPECIAL TOPIC Molecular Imaging in Nanotechnology and Theranostics Chrysafis Andreou,1 Suchetan Pal,1 Lara Rotter,2 Jiang Yang,1 Moritz F. Kircher1,3,4 1Department of Radiology, Memorial Sloan Kettering Cancer Center, New York, NY, 10065, USA 2Department of Neurology, Memorial Sloan Kettering Cancer Center, New York, NY, 10065, USA 3Center for Molecular Imaging and Nanotechnology (CMINT), Memorial Sloan Kettering Cancer Center, New York, NY, 10065, USA 4Department of Radiology, Weill Cornell Medical College, New York, NY, 10065, USA Abstract The fields of biomedical nanotechnology and theranostics have enjoyed exponential growth in recent years. The BMolecular Imaging in Nanotechnology and Theranostics^ (MINT) Interest Group of the World Molecular Imaging Society (WMIS) was created in order to provide a more organized and focused forum on these topics within the WMIS and at the World Molecular Imaging Conference (WMIC). The interest group was founded in 2015 and was officially inaugurated during the 2016 WMIC. The overarching goal of MINT is to bring together the many scientists who work on molecular imaging approaches using nanotechnology and those that work on theranostic agents. MINT therefore represents scientists, labs, and institutes that are very diverse in their scientific backgrounds and areas of expertise, reflecting the wide array of materials and approaches that drive these fields. In this short review, we attempt to provide a condensed overview over some of the key areas covered by MINT. Given the breadth of the fields and the given space constraints, we have limited the coverage to the realm of nanoconstructs, although theranostics is certainly not limited to this domain.