Absorption Spectroscopy
Total Page:16
File Type:pdf, Size:1020Kb
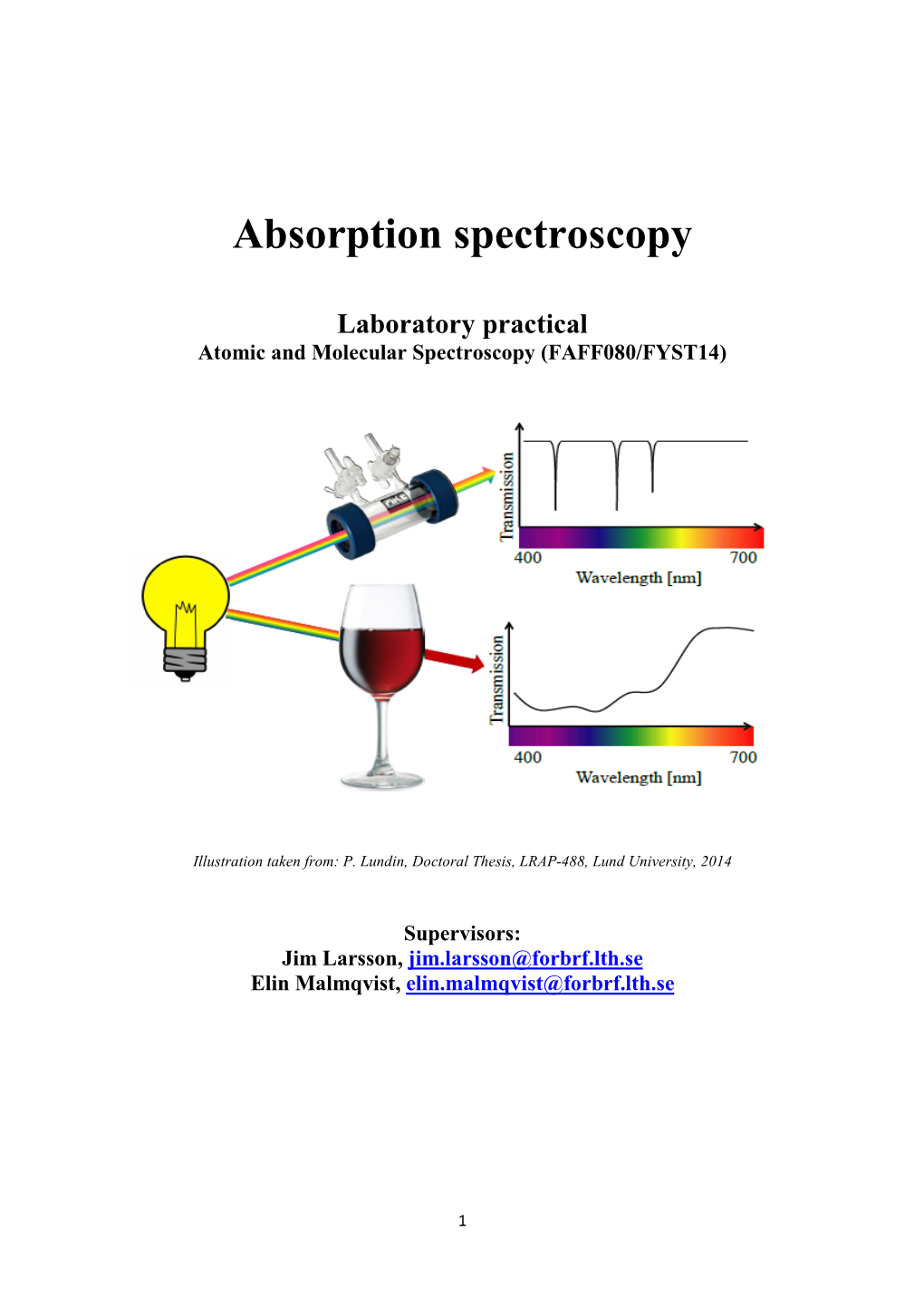
Load more
Recommended publications
-
Lecture 6: Spectroscopy and Photochemistry II
Lecture 6: Spectroscopy and Photochemistry II Required Reading: FP Chapter 3 Suggested Reading: SP Chapter 3 Atmospheric Chemistry CHEM-5151 / ATOC-5151 Spring 2005 Prof. Jose-Luis Jimenez Outline of Lecture • The Sun as a radiation source • Attenuation from the atmosphere – Scattering by gases & aerosols – Absorption by gases • Beer-Lamber law • Atmospheric photochemistry – Calculation of photolysis rates – Radiation fluxes – Radiation models 1 Reminder of EM Spectrum Blackbody Radiation Linear Scale Log Scale From R.P. Turco, Earth Under Siege: From Air Pollution to Global Change, Oxford UP, 2002. 2 Solar & Earth Radiation Spectra • Sun is a radiation source with an effective blackbody temperature of about 5800 K • Earth receives circa 1368 W/m2 of energy from solar radiation From Turco From S. Nidkorodov • Question: are relative vertical scales ok in right plot? Solar Radiation Spectrum II From F-P&P •Solar spectrum is strongly modulated by atmospheric scattering and absorption From Turco 3 Solar Radiation Spectrum III UV Photon Energy ↑ C B A From Turco Solar Radiation Spectrum IV • Solar spectrum is strongly O3 modulated by atmospheric absorptions O 2 • Remember that UV photons have most energy –O2 absorbs extreme UV in mesosphere; O3 absorbs most UV in stratosphere – Chemistry of those regions partially driven by those absorptions – Only light with λ>290 nm penetrates into the lower troposphere – Biomolecules have same bonds (e.g. C-H), bonds can break with UV absorption => damage to life • Importance of protection From F-P&P provided by O3 layer 4 Solar Radiation Spectrum vs. altitude From F-P&P • Very high energy photons are depleted high up in the atmosphere • Some photochemistry is possible in stratosphere but not in troposphere • Only λ > 290 nm in trop. -
Photon Cross Sections, Attenuation Coefficients, and Energy Absorption Coefficients from 10 Kev to 100 Gev*
1 of Stanaaros National Bureau Mmin. Bids- r'' Library. Ml gEP 2 5 1969 NSRDS-NBS 29 . A111D1 ^67174 tioton Cross Sections, i NBS Attenuation Coefficients, and & TECH RTC. 1 NATL INST OF STANDARDS _nergy Absorption Coefficients From 10 keV to 100 GeV U.S. DEPARTMENT OF COMMERCE NATIONAL BUREAU OF STANDARDS T X J ". j NATIONAL BUREAU OF STANDARDS 1 The National Bureau of Standards was established by an act of Congress March 3, 1901. Today, in addition to serving as the Nation’s central measurement laboratory, the Bureau is a principal focal point in the Federal Government for assuring maximum application of the physical and engineering sciences to the advancement of technology in industry and commerce. To this end the Bureau conducts research and provides central national services in four broad program areas. These are: (1) basic measurements and standards, (2) materials measurements and standards, (3) technological measurements and standards, and (4) transfer of technology. The Bureau comprises the Institute for Basic Standards, the Institute for Materials Research, the Institute for Applied Technology, the Center for Radiation Research, the Center for Computer Sciences and Technology, and the Office for Information Programs. THE INSTITUTE FOR BASIC STANDARDS provides the central basis within the United States of a complete and consistent system of physical measurement; coordinates that system with measurement systems of other nations; and furnishes essential services leading to accurate and uniform physical measurements throughout the Nation’s scientific community, industry, and com- merce. The Institute consists of an Office of Measurement Services and the following technical divisions: Applied Mathematics—Electricity—Metrology—Mechanics—Heat—Atomic and Molec- ular Physics—Radio Physics -—Radio Engineering -—Time and Frequency -—Astro- physics -—Cryogenics. -
Review and History of Photon Cross Section Calculations*
INSTITUTE OF PHYSICS PUBLISHING PHYSICS IN MEDICINE AND BIOLOGY Phys. Med. Biol. 51 (2006) R245–R262 doi:10.1088/0031-9155/51/13/R15 REVIEW Review and history of photon cross section calculations* J H Hubbell National Institute of Standards and Technology, Ionizing Radiation Division, Mail Stop 8463, 100 Bureau Drive, Gaithersburg, MD 20899-8463, USA E-mail: [email protected] Received 22 February 2006, in final form 12 April 2006 Published 20 June 2006 Online at stacks.iop.org/PMB/51/R245 Abstract Photon (x-ray, gamma-ray, bremsstrahlung) mass attenuation coefficients, µ/ρ, are among the most widely used physical parameters employed in medical diagnostic and therapy computations, as well as in diverse applications in other fields such as nuclear power plant shielding, health physics and industrial irradiation and monitoring, and in x-ray crystallography. This review traces the evolution of this data base from its empirical beginnings totally derived from measurements beginning in 1907 by Barkla and Sadler and continuing up through the 1935 Allen compilation (published virtually unchanged in all editions up through 1971–1972 of the Chemical Rubber Handbook), to the 1949 semi-empirical compilation of Victoreen, as our theoretical understanding of the constituent Compton scattering, photoabsorption and pair production interactions of photons with atoms became more quantitative. The 1950s saw the advent of completely theoretical (guided by available measured data) systematic compilations such as in the works of Davisson and Evans, and by White-Grodstein under the direction of Fano, using mostly theory developed in the 1930s (pre-World War II) by Sauter, Bethe, Heitler and others. -
Rayleigh & Mie Scattering Cross Section Calculations And
Rayleigh & Mie scattering cross section calculations and implications for weather radar observations 1 Learning Objectives The following are the learning objectives for this assignment: • Familiarize yourself with some basic concepts related to radio wave scattering, absorption, and radar cross sections • Become acquainted with some of the fundamental definitions and foundations connected with the Mie theory • Explore under what conditions the Rayleigh approximation can be applied and to what extent it accurately represents radio wave scatter and absorption • Study the dependence of various radar cross sections on wavelength and temperature 2 Introduction As electromagnetic radiation propagates though our atmosphere, it interacts with air molecules, dust particles, water vapor, rain, ice particles, insects, and a host of other entities. These interac- tions result primarily in the form of scatter and absorption, both of which are important for remote sensing studies of the atmosphere. Typically, the degree to which a “target” can scatter or absorb electromagnetic radiation is described through its cross section σ. When a target is illuminated by a wave having a power density (irradiance) given by Si, it will scatter/absorb a portion of the wave. The cross section represents an apparent area, used to describe by what amount the radiation interacts with the target. An observer located at a particular location (described by θ and φ with respect to the wave’s propagation vector) will be detect radiation scattered by the target with a power density given by by Sr. Assuming that the target has scattered the incident electromagnetic radiation isotropically, then the cross section σ can be directly calculated using S (θ; φ) σ(θ; φ) = 4πr2 r ; (1) Si where r is the distance between the target and the observer. -
Barrett, App.C
81CHAEL J. fL Yl8 Radiological Itnaging The Theory of Image Formation, Detection, and Processing Volume 1 Harrison H. Barrett William Swindell Department of Radiology and Optical Sciences Center University of Arizona Tucson, Arizona @ 1981 ACADEMIC PRESS A Subsidiaryof Harcourt Bracejovanovich, Publishers New York London Paris San Diego San Francisco Sao Pau.lo Sydney Tokyo Toronto Appendix C Interaction of Photonswith Matter In this appendixwe briefly reviewthe interactionof x rays with matter. For more detailsthe readershould consult a standardtext suchas Heitler (1966) or Evans (1968). In Sections C.1-C.6 we consider matter in elemental form only. The extension to mixtures and compounds is outlined in Section C.7. C.1 ATTENUATION, SCATTERING, AND ABSORPTION When a primary x-ray beam passesthrough matter, it becomesweaker or attenuated as photons are progressively removed from it. This attenuation takes place by two competing processes:scattering and absorption.For our purposes, which involve diagnostic energy x rays and low-atomic-number elements, the distinction between scattering losses and absorption losses is clear. Scattering lossesrefer to the energy removed from the primary beam by photons that are redirected by (mainly Compton) scattering events.The energy is carried away from the site of the primary interaction. Absorption lossesrefer to the energy removed from the primary beam and transferred locally to the lattice in the form of heat. Absorbed energy is derived from the photoelectron in photoelectric interactions and from the recoil electron in Compton events. Energy that is lost from the primary flux by other than Compton scatteredradiation may neverthelessultimately appear as scattered radiation in the form of bremsstrahlung, k-fluorescence, or annihilation gamma rays. -
Module 2: Reactor Theory (Neutron Characteristics)
DOE Fundamentals NUCLEAR PHYSICS AND REACTOR THEORY Module 2: Reactor Theory (Neutron Characteristics) NUCLEAR PHYSICS AND REACTOR THEORY TABLE OF CONTENTS Table of Co nte nts TABLE OF CONTENTS ................................................................................................... i LIST OF FIGURES .......................................................................................................... iii LIST OF TABLES ............................................................................................................iv REFERENCES ................................................................................................................ v OBJECTIVES ..................................................................................................................vi NEUTRON SOURCES .................................................................................................... 1 Neutron Sources .......................................................................................................... 1 Intrinsic Neutron Sources ............................................................................................. 1 Installed Neutron Sources ............................................................................................ 3 Summary...................................................................................................................... 4 NUCLEAR CROSS SECTIONS AND NEUTRON FLUX ................................................. 5 Introduction ................................................................................................................. -
Absorption Cross-Sections of Ozone in the Ultraviolet And
Journal of Molecular Spectroscopy 327 (2016) 105–121 Contents lists available at ScienceDirect Journal of Molecular Spectroscopy journal homepage: www.elsevier.com/locate/jms Absorption cross-sections of ozone in the ultraviolet and visible spectral regions: Status report 2015 ⇑ Johannes Orphal a, , Johannes Staehelin b, Johanna Tamminen c, Geir Braathen d, Marie-Renée De Backer e, Alkiviadis Bais f, Dimitris Balis f, Alain Barbe e, Pawan K. Bhartia g, Manfred Birk h, James B. Burkholder aa, Kelly Chance j, Thomas von Clarmann a, Anthony Cox k, Doug Degenstein l, Robert Evans i, Jean-Marie Flaud m, David Flittner n, Sophie Godin-Beekmann o, Viktor Gorshelev p, Aline Gratien m, Edward Hare q, Christof Janssen r, Erkki Kyrölä c, Thomas McElroy s, Richard McPeters g, Maud Pastel o, Michael Petersen t,1, Irina Petropavlovskikh i,ab, Benedicte Picquet-Varrault m, Michael Pitts n, Gordon Labow g, Maud Rotger-Languereau e, Thierry Leblanc u, Christophe Lerot v, Xiong Liu j, Philippe Moussay t, Alberto Redondas w, Michel Van Roozendael v, Stanley P. Sander u, Matthias Schneider a, Anna Serdyuchenko p, Pepijn Veefkind x, Joële Viallon t, Camille Viatte y, Georg Wagner h, Mark Weber p, Robert I. Wielgosz t, Claus Zehner z a Institute for Meteorology and Climate Research (IMK), Karlsruhe Institute of Technology (KIT), Karlsruhe, Germany b Swiss Federal Institute of Technology (ETH), Zurich, Switzerland c Finnish Meteorological Institute (FMI), Helsinki, Finland d World Meteorological Organization (WMO), Geneva, Switzerland e GSMA, CNRS and University -
Arxiv:1605.03945V2 [Physics.Optics] 18 Jan 2017 Maximum Absorption Cross Section Cabs (At Resonance) Ground Are Given in a Supplemental Material (SM)
Fundamental Limits of Optical Force and Torque 1 1,2 1,3 1,3 A. Rahimzadegan∗ , R. Alaee∗ , I. Fernandez-Corbaton , and C. Rockstuhl 1Institute of Theoretical Solid State Physics, Karlsruhe Institute of Technology, Karlsruhe, Germany 2Max Planck Institute for the Science of Light, Erlangen, Germany 3Institute of Nanotechnology, Karlsruhe Institute of Technology, Karlsruhe, Germany ∗Equally contributed to the work. Corresponding authors: [email protected], [email protected] Optical force and torque provide unprecedented control on the spatial motion of small particles. A valid scientific question, that has many practical implications, concerns the existence of fundamental upper bounds for the achievable force and torque exerted by a plane wave illumination with a given intensity. Here, while studying isotropic particles, we show that different light-matter interaction channels contribute to the exerted force and torque; and analytically derive upper bounds for each of the contributions. Specific examples for particles that achieve those upper bounds are provided. We study how and to which extent different contributions can add up to result in the maximum optical force and torque. Our insights are important for applications ranging from molecular sorting, particle manipulation, nanorobotics up to ambitious projects such as laser-propelled spaceships. PACS numbers: 42.25.-p, 42.70.-a, 78.20.Bh, 78.67.Bf,42.50.Wk,42.50.Tx Optical scattering, extinction, and absorption cross cations and implications of the optical force and torque sections characterize the strength of light-matter- in many areas. Examples are the opto-mechanical ma- interaction. They quantify the fraction of power a par- nipulation14–17, molecular or particle optical sorting18, ticle scatters, extincts, or absorbs1,2. -
Thermal Neutron Capture Cross Sections Resonance Integrals and G-Factors
International Atomic Energy Agency INDC(NDS)-440 Distr. PG+R I N D C INTERNATIONAL NUCLEAR DATA COMMITTEE THERMAL NEUTRON CAPTURE CROSS SECTIONS RESONANCE INTEGRALS AND G-FACTORS S.F. Mughabghab Brookhaven National Laboratory Upton, NY 11973-5000 U.S.A. Research carried out under the auspices of the U.S. Department of Energy under Prime Contract No. DE-AC02-98CH10886, and under IAEA contract No 11376/USA February 2003 IAEA NUCLEAR DATA SECTION, WAGRAMER STRASSE 5, A-1400 VIENNA Reproduced by the IAEA in Austria February 2003 INDC(NDS)-440 Distr. PG+R THERMAL NEUTRON CAPTURE CROSS SECTIONS RESONANCE INTEGRALS AND G-FACTORS S.F. Mughabghab Brookhaven National Laboratory Upton, NY 11973-5000 U.S.A. Research carried out under the auspices of the U.S. Department of Energy under Prime Contract No. DE-AC02-98CH10886, and under IAEA contract No 11376/USA Abstract The thermal radiative capture cross sections and resonance integrals of elements and isotopes with atomic numbers from 1 to 83 (as well as 232Th and 238U) have been re-evaluated by taking into consideration all known pertinent data published since 1979. This work has been undertaken as part of an IAEA co-ordinated research project on "Prompt capture gamma-ray activation analysis". Westcott g-factors for radiative capture cross sections at a temperature of 300K were computed by utilizing the INTER code and ENDF-B/VI (Release 8) library files. The temperature dependence of the Westcott g-factor is illustrated for 113Cd , 124Xe and 157Gd at temperatures of 150, 294 and 400K. Comparisons have also been made of the newly evaluated capture cross sections of 6Li, 7Li, 12C and 207Pb with those determined by the k0 method. -
Critical Review of Ultraviolet Photoabsorption Cross Sections for Molecules of Astrophysical and Aeronomic Interest
NATL INST OF STANDARDS & TECH R.I.C. All 101 787744 /NSRDS-NBS QC100 .U573 V38;1971 C.1 NBS-PUB-R 1964 NSRDS—NBS 38 Critical Review of Ultraviolet - Photoabsorption Cross Sections for Molecules of Astrophysical and Aeronomic Interest u.s. DEPARTMENT OF COMMERCE National Bureau of ndards — NATIONAL BUREAU OF STANDARDS 1 The National Bureau of Standards was established by an act of Congress March 3, 1901. The Bureau’s overall goal is to strengthen and advance the Nation’s science and technology and facilitate their effective application for public benefit. To this end, the Bureau conducts research and provides: (1) a basis for the Nation’s physical measure- ment system, (2) scientific and technological services for industry and government, (3) a technical basis for equity in trade, and (4) technical services to promote public safety. The Bureau consists of the Institute for Basic Standards, the Institute for Materials Research, the Institute for Applied Technology, the Center for Computer Sciences and Technology, and the Office for Information Programs. THE INSTITUTE FOR BASIC STANDARDS provides the central basis within the United States of a complete and consistent system of physical measurement; coordinates that system with measurement systems of other nations; and furnishes essential services leading to accurate and uniform physical measurements throughout the Nation’s scien- tific community, industry, and commerce. The Institute consists of a Center for Radia- tion Research, an Office of Measurement Services and the following divisions: Applied Mathematics—Electricity—Heat—Mechanics—Optical Physics—Linac Radiation 2—Nuclear Radiation 2—Applied Radiation 2—Quantum Electronics 3— Electromagnetics 3—Time and Frequency 3—Laboratory Astrophysics 3—Cryo- 3 genics . -
Cross-Section of Single-Crystal Materials Used As Thermal Neutron Filters
VII Radiation Physics & Protection Conference, 27-30 November 2004,Ismailia-Egypt EG0500275 Cross-Section of Single-Crystal Materials used as Thermal Neutron Filters M.Adib Reactor Physics Dept. NRC, AEA Cairo Egypt Email Adibmamdouh @yahoo.com ABSTRACT Transmission properties of several single crystal materials important for neutron scattering instrumentation are presented. A computer codes are developed which permit the calculation of thermal diffuse and Bragg-scattering cross-sections of silicon., and sapphire as a function of material’s constants, temperature and neutron energy, E, in the range 0.1 meV .A discussion of the use of their single-crystal as a thermal neutron filter in terms of the optimum crystal thickness, mosaic spread, temperature, cutting plane and tuning for efficient transmission of thermal-reactor neutrons is given. Keywords: Thermal Neutron Filters,Sapphire,Silicon INTRODUCTION The use of large, perfect single-crystals of various materials as filters for thermal neutron beams has long been known [1]. Several materials such as quartz (SiO2) [2], bismuth [3], silicon [4], lead [5] and sapphire (Al2O3) [6-9] have been suggested as most successful filter materials. At high neutron energies, greater than about 1eV, the total neutron cross section σt of each of the above mentioned materials is in the range of a few barns, but at lower thermal energies, less than 0.1eV, where much of the coherent Bragg scattering is disallowed the effective cross-section for single-crystal specimens is much reduced. That is also due to the decrease of the thermal diffuse (TDS) or inelastic scattering cross-section with the decrease of neutron energy. -
Tables of X-Ray Mass Attenuation Coefficients and Mass Energy-Absorption Coefficients 1 Kev to 20 Mev for Elements Z = 1 to 92 A
NAT'L INST. OF STAND & TECH III III III Hill HI AlllOb 243004 NIST RESEARCH INFORMATION NISTIR 5632 NIST MAY 1 6 1995 PUBLICATIONS 1 CENTER Tables of X-Ray Mass Attenuation Coefficients and Mass Energy-Absorption Coefficients 1 keV to 20 MeV for Elements Z = 1 to 92 and 48 Additional Substances of Dosimetric Interest J. H. Hubbell S. M. Seltzer U.S. DEPARTMENT OF COMMERCE Technology Administration National Institute of Standards and Technology Physics Laboratory Ionizing Radiation Division Gaithersburg, MD 20899 qo. \n> • fUlO NIST NISTIR 5632 Tables of X-Ray Mass Attenuation Coefficients and Mass Energy-Absorption Coefficients 1 keV to 20 MeV for Elements Z = 1 to 92 and 48 Additional Substances of Dosimetric Interest J. H. Hubbell S. M. Seltzer U.S. DEPARTMENT OF COMMERCE Technology Administration National Institute of Standards and Technology Physics Laboratory Ionizing Radiation Division Gaithersburg, MD 20899 May 1995 U.S. DEPARTMENT OF COMMERCE Ronald H. Brown, Secretary TECHNOLOGY ADMINISTRATION Mary L. Good, Under Secretary for Technology NATIONAL INSTITUTE OF STANDARDS AND TECHNOLOGY Arati Prabhakar, Director TABLES OF X-RAY MASS ATTENUATION COEFFICIENTS AND MASS ENERGY- ABSORPTION COEFFICIENTS 1 keV TO 20 MeV FOR ELEMENTS Z = 1 TO 92 AND 48 ADDITIONAL SUBSTANCES OF DOSIMETRIC INTEREST* + J. H. Hubbell 11830 Rocking Horse Road, Rockville, MD 20852 and S. M. Seltzer Ionizing Radiation Division, Physics Laboratory National Institute of Standards and Technology Technology Administration, U.S. Department of Commerce Gaithersburg, MD 20899 U.S. A. Abstract Tables and graphs of the photon mass attenuation coefficient p/p and the mass = 1 energy-absorption coefficient pen/p are presented for all of the elements Z to 92, and for 48 compounds and mixtures of radiological interest.