Safety Analysis of Embankment Dams and Alternative
Total Page:16
File Type:pdf, Size:1020Kb
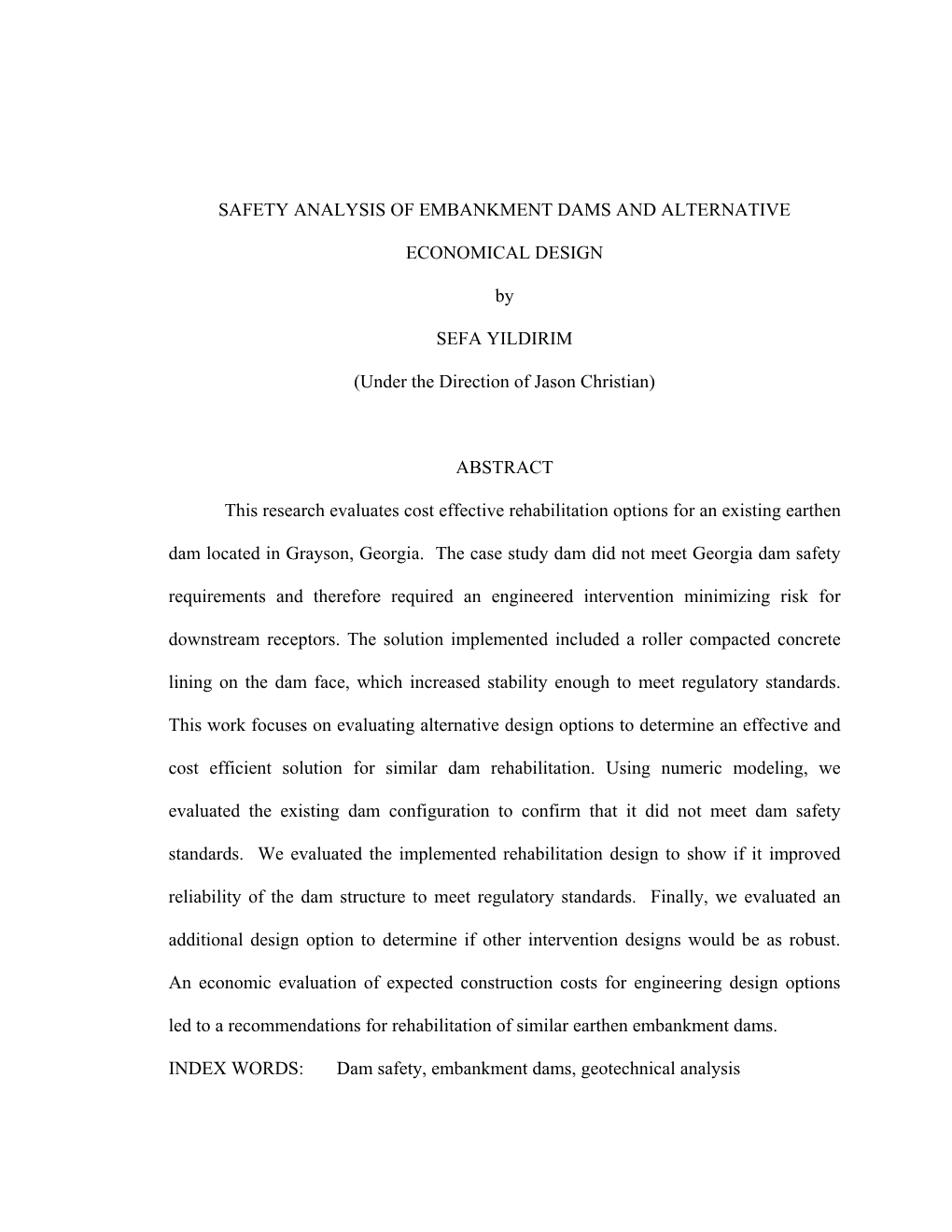
Load more
Recommended publications
-
Strasbourg, 4 Mars 2011 AP/CAT (2011) 05Rev
1 Strasbourg, 4 Mars 2011 AP/CAT (2011) 05rev ACCORD EUROPEEN ET MEDITERRANEEN EUROPEAN AND MEDITERRANEEN SUR LES RISQUES MAJEURS MAJOR HAZARDS AGREEMENT (EUR-OPA) (EUR-OPA) RESEAU DES CENTRES EURO-MEDITERRANEENS SPECIALISES DE L'ACCORD EUR-OPA RISQUES MAJEURS PROGRAMMES COORDONNES EN 2010 NETWORK OF SPECIALISED EURO-MEDITERRANEAN CENTRES OF THE EUR-OPA MAJOR HAZARDS AGREEMENT 2010 COORDINATED PROGRAMMES www.coe.int/europarisks 2 TABLE DES MATIERES / CONTENTS NATIONAL AND MUNICIPAL CAMPAIGNS ON INFORMING AND WARNING THE POPULATION ABOUT EMERGENCIES AT CENTRAL AND MUNICIPAL LEVELS: BASIS FOR A REGIONAL EARLY WARNING SYSTEM FOR SOUTHERN CAUCASIAN COUNTRIES AND NEIGHBOURING STATES IN TRANS-FRONTIER EMERGENCIES (ECRM - European Interregional Scientific And Educational Centre On Major Risk Management, Yerevan) ..3 COASTLINE AT RISK: METHODS FOR MULTI-HAZARD ASSESSMENT (CERG - European Centre For Seismic And Geomorphological Hazards, Strasbourg) ..............................................................................................8 DAM RELATED RISKS IN EUROPE AND THE MEDITERRANEAN: THREATS AND PREVENTION (GHHD - Geodynamical Hazards Of High Dams, Tbilisi) ..................................................................................... 13 FORMATION CONNAISSANCE ET GESTION DES RISQUES COTIERS (CERCO - Centre Europeen Sur Les Risques Cotiers, Biarritz) .............................................................................................................................33 ECGS-FKPE WORKSHOP INDUCED SEISMICITY (ECGS - European -
The Gilboa Dam and Its Possible Failure: What It Means for Nyc and Upstate New York
CORE Metadata, citation and similar papers at core.ac.uk Provided by Union College: Union | Digital Works Union College Union | Digital Works Honors Theses Student Work 2008 The iG lboa Dam and its possible failure: what it means for NYC and Upstate New York Kelly C. Owens Follow this and additional works at: https://digitalworks.union.edu/theses Part of the Natural Resources Management and Policy Commons, and the Water Resource Management Commons Recommended Citation Owens, Kelly C., "The iG lboa Dam and its possible failure: what it means for NYC and Upstate New York" (2008). Honors Theses. 1574. https://digitalworks.union.edu/theses/1574 This Open Access is brought to you for free and open access by the Student Work at Union | Digital Works. It has been accepted for inclusion in Honors Theses by an authorized administrator of Union | Digital Works. For more information, please contact [email protected]. THE GILBOA DAM AND ITS POSSIBLE FAILURE: WHAT IT MEANS FOR NYC AND UPSTATE NEW YORK By Kelly C. Owings * * * * * * * * * Submitted in partial fulfillment of the requirements for Honors in the Department of Environmental Studies UNION COLLEGE June, 2008 ABSTRACT The majority of the NYC water supply is provided by a system of dams and reservoirs that have been created in upstate New York. As the owner of this water supply system, NYC is responsible for managing each of the dams and reservoirs within the system. This responsibility includes upholding dam regulations in order to ensure the safety of the citizens of the surrounding area and to lesson any environmental impacts that may occur due to the water supply system. -
What We Do and Don't Know About Alabama's Dams and Why It Matters! 2017 ASCE Alabama Section Winter Meeting March 2, 2017
What we do and don't know about Alabama's dams and why it matters! 2017 ASCE Alabama Section Winter Meeting March 2, 2017 Clay Campbell, PE Amec Foster Wheeler Environment and Infrastructure, Inc. Birmingham, AL Safety Moment - Oroville Dam Background Issue ►Earthen embankment ►Crater formed in main ►770 feet tall spillway ►Tallest dam in U.S. ►Emergency spillway activated and eroded ►Used for: ► Water supply ►188,000 residents ► Hydroelectricity evacuated ► Flood control ► Storage = 3.5 million acre-feet ► Completed in 1968 2 Problem statement ► “Of the large dams 85 percent ► More than 600 dams need to be would have exceeded their upgraded to ensure the safety of design lifespan by 2020 or soon those downstream (NRCS-small thereafter” (Federal Emergency watershed projects) Management 2001). Hazard classification criteria Based on the National Engineering Manual (NEM) ► High Hazard – Class C – This is a dam where failure may cause loss of life or serious damage to homes, industrial and commercial buildings, important public utilities, main highways, or railroads. ► Significant Hazard – Class B – This is a dam in a predominantly rural or agricultural area where failure may damage isolated homes, main highways, or minor railroads or interrupt service of relatively important public utilities. ► Low Hazard – Class A – This is a dam that is located in rural or agricultural areas where failure may damage farm buildings, agricultural land, or township and country roads. Alabama dams National Inventory of Dams ► 2,271 dams in Alabama ► # is growing with inventory being put together by the State of Alabama Office of Water Resources 5 Alabama dams Pre-1965 = over 50 years old 6 National Inventory of Dams Alabama dams 7 National Inventory of Dams Alabama dams 8 National Inventory of Dams Alabama dams 9 National Inventory of Dams AL OWR dam inventory update Draft results: ► 5,209 total dams ► 209 high hazard ► 1,127 significant hazard ► 3,873 low hazard ► Only 1,510 of the NID points accurately represent an actual dam. -
Dam Removal Success Stories
DAM REMOVAL SUCCESS STORIES R ESTORING R IVERS THROUGH S ELECTIVE R EMOVAL OF D AMS THAT D ON’ T M AKE S ENSE DECEMBER 1999 DAM REMOVAL SUCCESS STORIES R ESTORING R IVERS THROUGH S ELECTIVE R EMOVAL OF D AMS THAT D ON’ T M AKE S ENSE DECEMBER 1999 This report was prepared by Friends of the Earth, American Rivers, and Trout Unlimited in December 1999. Cover Photographs: Sandstone Dam on the Kettle River in Minnesota Source: Ian Chisholm, Minnesota Department of Natural Resources Cover Design: Gallagher/Wood Design Text Design: American Rivers (c) December 1999 by American Rivers, Friends of the Earth, & Trout Unlimited. All Rights Reserved. ISBN 0-913890-96-0 Printed with soy ink on 100% recycled, 30% post consumer waste, chlorine free paper. Dam Removal Success Stories Final Report iii ACKNOWLEDGEMENTS Edited by Elizabeth Maclin and Matt Sicchio of American Rivers, with contributions from American Rivers staff Margaret Bowman, Steve Brooke, Jocelyn Gibbon and Amy Souers; Friends of the Earth staff Brent Blackwelder, Shawn Cantrell and Lisa Ramirez; and Trout Unlimited staff Brian Graber, Sara Johnson and Karen Tuerk. Thanks also to the following individuals for their assistance in the research and writing phase of this publication: Ian Chisholm, Karen Cozzetto, David Cummings, John Exo, Bob Hunter, Lance Laird, Stephanie Lindloff, David Morrill, Richard Musgrove, Dirk Peterson, Tom Potter, Craig Regalia, Elizabeth Ridlington, Jed Volkman, Bob Wengrzynek and Laura Wildman. Special thanks to the many local contacts (listed in each case study) who gave so generously of their time, and without whom this report would not have been possible. -
RCEM – Reclamation Consequence Estimating Methodology
RCEM – Reclamation Consequence Estimating Methodology Interim – DRAFT Dam Failure and Flood Event Case History Compilation Lawn Lake Dam failure flood in 1982 at Estes Park, CO (Reclamation photograph) U.S. Department of the Interior Bureau of Reclamation February 2014 Mission Statements The U.S. Department of the Interior protects America’s natural resources and heritage, honors our cultures and tribal communities, and supplies the energy to power our future. The mission of the Bureau of Reclamation is to manage, develop, and protect water and related resources in an environmentally and economically sound manner in the interest of the American public. Interim – DRAFT RCEM – Reclamation Consequence Estimating Methodology Dam Failure and Flood Event Case History Compilation U.S. Department of the Interior Bureau of Reclamation February 2014 RCEM – Case History Compilation Interim – DRAFT Table of Contents List of Figures ......................................................................................................................... iii Introduction .............................................................................................................................. 1 High Severity Dam Failure and Flooding Case Histories ..................................................... 4 Vega de Tera Dam - Failed January 9, 1959 ....................................................................... 4 St. Francis Dam – Failed March 12-13, 1928 ...................................................................... 6 Nevado del Ruiz -
Breaching Charateristics of Dam Failures
BREACHING CHARACTERISTICS OF DAM FAILURES By Thomas C. MacDonald1 and Jennifer Langridge-Monopolis2 AiSTRACT: Computer programs developed for dam safety analyses are limited by the accuracy of the input data for the geometric and temporal dam breach characteristics. Data on a number of historical dam failures were collected and analyzed and graphical relationships for predicting breach characteristics were developed for erosion type breaches. The data provides a basis for selecting a breach shape and calculating the breach size and the time for breach devel opment. A relationship is also developed for estimating peak outflows from dam failures. This relationship can be used to verify the methodology and the results of dam safety studies. INTRODUCTION In recent years significant effort has been directed at determining the safety of dams in the United States and abroad. One aspect of dam safety is the potential for loss of life and damages in the downstream flood- plain that would result in the event of a dam failure. To assess the po tential hazards of dam failures, sophisticated computer programs have been developed that simulate dam break hydrographs, and route these hydrographs downstream so that inundated areas, flow depths, and flow velocities can be estimated. Two of the commonly used computer pro grams for dam break analyses are the U.S. Army Corps of Engineers' HEC-1 program and the U.S. National Weather Service's program en titled DAMBRK. Although the available computer programs utilize state-of-the-art hy- drograph development and routing techniques, they are dependent on certain inputs regarding the geometric and temporal characteristics of the dam breach. -
Dams Sector: Estimating Loss of Life for Dam Failure
Dams Sector Estimating Loss of Life for Dam Failure Scenarios September 2011 ii Contents Page Executive Summary v 1. Introduction 1 1.1 Dam Failures in the United States 2 2. Methods for Estimating Loss of Life Resulting from Dam Failure 6 2.1 Key Concepts and Considerations in Estimating Loss of Life from a Dam Failure 6 2.2 Model Limitations and Uncertainty of Results 7 2.3 Data Requirements and Sources 8 2.4 Bureau of Reclamation DSO-99-06 Procedure 9 2.4.1 Advantages of the DSO-99-06 Procedure 10 2.4.2 Limitations of the DSO-99-06 Procedure 11 2.4.3 Application of the DSO-99-06 Procedure 12 2.5 Flood Comparison Method 25 2.5.1 Advantages and Strengths of the Flood Comparison Method 26 2.5.2 Limitations of the Flood Comparison Method 27 2.5.3 Application of the Flood Comparison Method 27 2.6 USACE/Utah State University LIFESim and Simplified LIFESim Models 30 2.6.1 LIFESim Model 30 2.6.2 Simplified LIFESim Model 35 2.6.3 Comparison of LIFESim and Simplified LIFESim 41 2.7 BC Hydro Life Safety Model 42 3. Current and Future Developments Related to Life-Loss Estimation 45 3.1 Determination of Flood Severity Zones Using 2D Modeling Results 45 3.2 Analysis-Based Efforts to Refine Flood Severity Categorization in Relation to Fatality Rates 46 3.3 Benchmark Studies and Model Enhancements 46 4. Application Example 48 4.1 DSO-99-06 Procedure 49 4.2 Flood Comparison Method 57 Acronym List 60 References 61 Appendix A: Synopsis of Notable United States Dam Failures 62 Mill River Dam, Massachusetts 62 South Fork Dam (Johnstown Flood), Pennsylvania 64 Walnut Grove Dam, Arizona 65 Austin Dam, Pennsylvania 66 iii St. -
National Dam Safety Program Research Needs Workshop: Embankment Dam Failure Analysis Preface
The National Dam Safety Program Research Needs Workshop: Embankment Dam Failure Analysis Preface One of the activities authorized by the Dam Safety and Security Act of 2002 is research to enhance the Nation’s ability to assure that adequate dam safety programs and practices are in place throughout the United States. The Act of 2002 states that the Director of the Federal Emergency Management Agency (FEMA), in cooperation with the National Dam Safety Review Board (Review Board), shall carry out a program of technical and archival research to develop and support: • improved techniques, historical experience, and equipment for rapid and effective dam construction, rehabilitation, and inspection; • devices for continued monitoring of the safety of dams; • development and maintenance of information resources systems needed to support managing the safety of dams; and • initiatives to guide the formulation of effective policy and advance improvements in dam safety engineering, security, and management. With the funding authorized by the Congress, the goal of the Review Board and the Dam Safety Research Work Group (Work Group) is to encourage research in those areas expected to make significant contributions to improving the safety and security of dams throughout the United States. The Work Group (formerly the Research Subcommittee of the Interagency Committee on Dam Safety) met initially in February 1998. To identify and prioritize research needs, the Subcommittee sponsored a workshop on Research Needs in Dam Safety in Washington D.C. in April 1999. Representatives of state and federal agencies, academia, and private industry attended the workshop. Seventeen broad area topics related to the research needs of the dam safety community were identified.