Resonance Capture of Neutrons by Uranium Cylinders
Total Page:16
File Type:pdf, Size:1020Kb
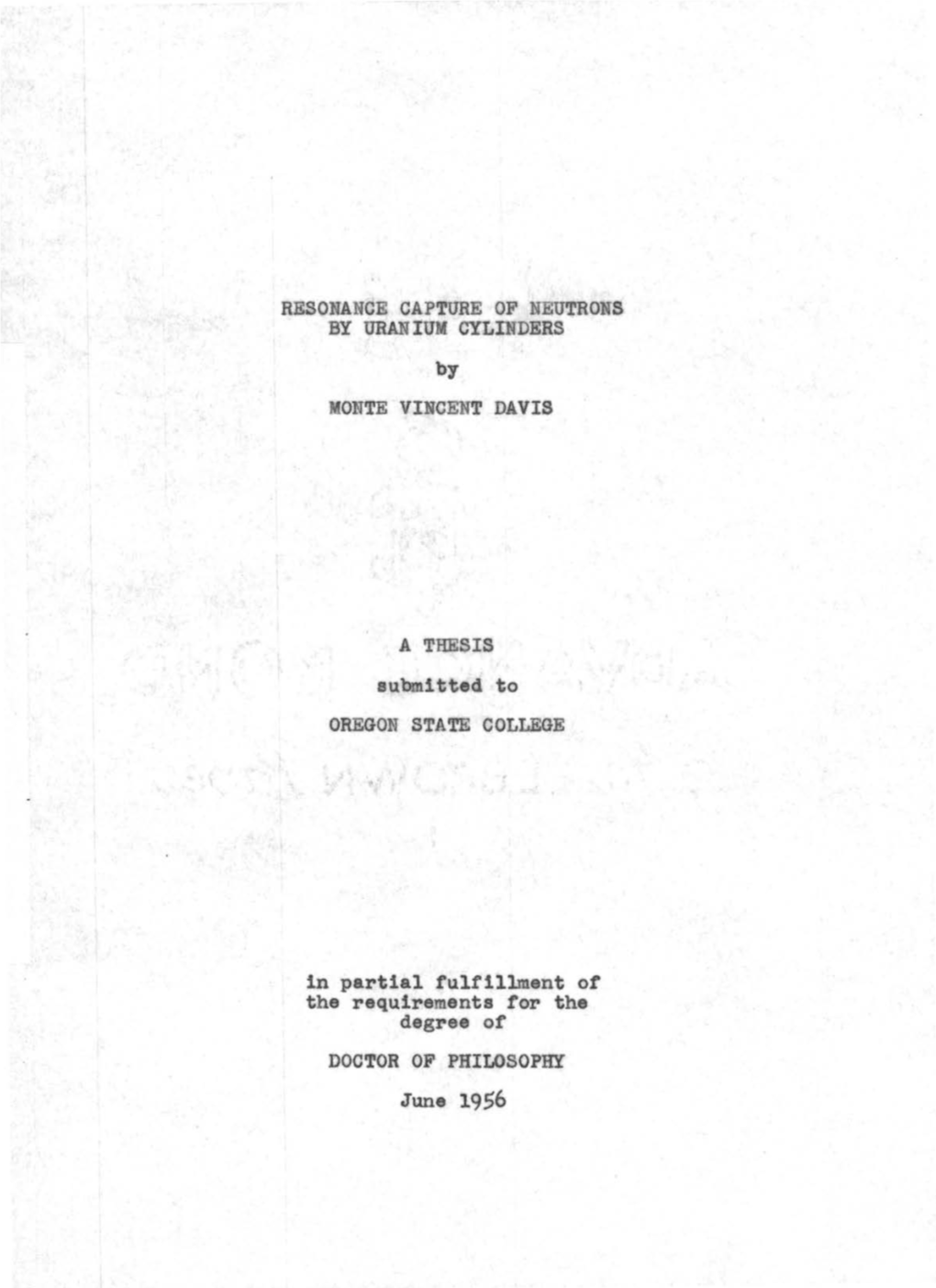
Load more
Recommended publications
-
Conceptual Design Report Jülich High
General Allgemeines ual Design Report ual Design Report Concept Jülich High Brilliance Neutron Source Source Jülich High Brilliance Neutron 8 Conceptual Design Report Jülich High Brilliance Neutron Source (HBS) T. Brückel, T. Gutberlet (Eds.) J. Baggemann, S. Böhm, P. Doege, J. Fenske, M. Feygenson, A. Glavic, O. Holderer, S. Jaksch, M. Jentschel, S. Kleefisch, H. Kleines, J. Li, K. Lieutenant,P . Mastinu, E. Mauerhofer, O. Meusel, S. Pasini, H. Podlech, M. Rimmler, U. Rücker, T. Schrader, W. Schweika, M. Strobl, E. Vezhlev, J. Voigt, P. Zakalek, O. Zimmer Allgemeines / General Allgemeines / General Band / Volume 8 Band / Volume 8 ISBN 978-3-95806-501-7 ISBN 978-3-95806-501-7 T. Brückel, T. Gutberlet (Eds.) Gutberlet T. Brückel, T. Jülich High Brilliance Neutron Source (HBS) 1 100 mA proton ion source 2 70 MeV linear accelerator 5 3 Proton beam multiplexer system 5 4 Individual neutron target stations 4 5 Various instruments in the experimental halls 3 5 4 2 1 5 5 5 5 4 3 5 4 5 5 Schriften des Forschungszentrums Jülich Reihe Allgemeines / General Band / Volume 8 CONTENT I. Executive summary 7 II. Foreword 11 III. Rationale 13 1. Neutron provision 13 1.1 Reactor based fission neutron sources 14 1.2 Spallation neutron sources 15 1.3 Accelerator driven neutron sources 15 2. Neutron landscape 16 3. Baseline design 18 3.1 Comparison to existing sources 19 IV. Science case 21 1. Chemistry 24 2. Geoscience 25 3. Environment 26 4. Engineering 27 5. Information and quantum technologies 28 6. Nanotechnology 29 7. Energy technology 30 8. -
22.4 Optimization of He-II UCN Source with Spallation Neutron Source
JP0150620 JAERI-Conf 2001-002 ICANS-XV 15th Meeting of the International Collaboration on Advanced Neutron Sources November 6-9,2000 Tsukuba, Japan 22.4 Optimization of He-II UCN Source with Spallation Neutron Source K. Mishima1, M. Ooi2, E. Choi1, Y. Kiyanagi2, Y. Masuda3*, S. Muto3, M. Tanaka4 and M. Yoshimura1 1 Research Center for Nuclear Physics, Osaka University, Ibaraki-shi, 567-0047 Japan 2 Hokkaido University, Sapporo-shi, 060-0808 Japan 3 KEK, 1-1 Oho, Tsukuba-shi, 305-0801 Japan 4 Kobe Tokiwa Collage, Kobe-shi, 653-0838 Japan * [email protected] Abstract A spallation neutron source was designed for super thermal UCN production in He-II. The configuration of neutron production target, moderator and He-II bottle was optimized in order to obtain high neutron flux with low y heating in He-II. In the optimization the advantage of the spallation neutron source is used: The spallation neutron source has high n/y ratio and freedom in target moderator configuration in comparison with the reactor. As a result, a great improvement in UCN density is expected compared with the present most intense UCN source at the Grenoble reactor. Introduction Ultra cold neutrons (UCNs) have been used for the measurements of /3 -decay lifetime, neutron electric dipole moment (EDM) and so on. UCNs have also a promising role in neutron p-decay asymmetry experiments. The experimental accuracy and - 1094 - JAERI-Conf 2001-002 possibility in these experiments are limited by UCN density. The motivation of the present work is to realize high intensity UCN source far beyond the Grenoble UCN source that is the most intense UCN source at present. -
Safety Aspects in Plutonium Handling
B.A.R.C./I-259 1 < n GOVERNMENT OF INDIA ATOMIC ENERGY COMMISSION SAFETY ASPBCTS IN PLUTONIUM HANDLING by S. Janardhanan, T. N. Krishnamurthi, S. B. Dabhadkar and S. D. Soman Health Physics Division BHABHA ATOMIC RESEARCH CENTRE BOMBAY, INDIA 1973 B.AJI.C./I-259 GOVERNMENT OF INDIA ATOMIC ENERGY COMISSION SA5BTY ASPECTS IN FTAJTONIUM HANDLING by S. Janardhanan, T.N. Krishnamurthl, S.D. Dabhadkar and S.D. Soman Health HiyBics Division BHABHA ATOMIC RESEARCH CENTRE BOMBAY, INDIA 1973 COMPEMPS fege So. 1. INTRODUCTION 1 2. RADIOLOGICAL SAFETY 1 2.1 Formation of ELutoniun iBotopes 1 2.2 Nuclear Characteristics of FLutoniua Radionuclides 2 2.3 External Hazardo 2 2.4 Internal Hazards 23 3. ENGINEERING SAFETY 31 "5.1 Glove Box for Hutoniun Handling 31 3.2 Design Safety Consideration 32 3.3 Ventilation for Hutonlum Laboratory 35 3.4 Surfaoe Finish for Plutonium Laboratory 36 3.5 Ere -commie 8 ioning Checks for Plutonium Laboratory 56 4. aONTAMINVTION EVALUATION, CONTROL AND HffiVENTION 37 4.1 Special Technique for Pu-Alr Monitoring 38 4i2 Surface and Bars onnel Monitoring 39 4.3 Decontamination 39 5. PLUTONIUM FIRE SAFETY 48 5.1 Plutonium Metal FireB . 49 5.2 Protective Measures for Hutonium Fires 50 5.3 Fire Safety in Storage, Handling and Shipment of 51 HLutonium Metal 6. CRITICALITY SAFETY . 51 6.1 Oriticality BarameterB 52 6.2 MethodB of Criticality Control 54 6.3 Safety FactorB 55 Page No. 6.4 Criticelity Data 57 6.5 Soluble Poisons 61 f, .6 Solid Poisons 61 f) .7 Pipe Intersections 61 6.8 Storage of Pu Metal, Compounds and Solution 61 6.9 Transport of Plutonium Metal, Compounds and Solution 68 6.10 Magnitude of Critieality Accident 68 6.11 Administration of Nuclear Safety 72 REFERENCES 73 Appendix-Is Design Details of a Typical Inert Atmosphere 75 Glove Box for Plutonium Handling laboratory Appendix-Hi Design Details of a Typical Normal Atmosphere 79 Glove Box and Pumehood SAFETY ASPECTS ITT PLUTONIUM HANDLING by S. -
Cross Sections and Neutron Yields for U-233, U-235 and Pu-239 at 2200
AE-11 Cross sections and neutron yields for U233, U235 and Pu239 at 2200 mjsec. N.G. Sjostrand andJ.S. Story AKTIEBOLAGET ATOMENERGI STOCKHOLM • SWEDEN • I960 ADDENDUM to report AE - 11 233 Cross sections and neutron yields for U and Pu239 at 2200 m/sec. N. Go Sjostrand and J. S. Story This is a preliminary report written in February 1959 and not intended for wide distribution. No corrections or additions have been made for this new edition. AE-11 Cross sections and neutron yields for U233, U235 and Pu239 at 2200 m/sec. N.G. Sjostrand and J.S. Story Summary: The experimental information on the 2200 m/sec values for <r , , ™ 233 235 239 3-ds (T^, a, V, and rj for U , U and Pu has been collected and discussed. The values will later be used in an evaluation of a "best" set of data. In an appendix the isotopic abundances of the uranium isotopes are discussed and 239 also the alpha activities of the uranium isotopes and Pu . Printed April 1960 LIST OF CONTENTS Page The absorption cross-sections 2 Notes on data in Table 3 4 The fission cross section 5 The capture to fission ratio 9 The number of neutrons per fission 11 The number of fission neutrons per absorbed neutron 14 Appendix. Half-lives and atomic abundances of the isotopes in natural uranium 20 Notation 20 Experimental Results. Mass spectrometry 20 Alpha Counting Experiments 21 Assessment of Recommended Values 26 References 29 TABLES 1. g-factors according to WESTCOTT (1958) 1 2. 2 3.0". -
TRIUMF Ultracold Neutron Source
TRIUMF Ultracold Neutron Source J.W. Martin (Spokesperson)1, L. Buchmann2, J.D. Bowman3, L. Clarke4, C. Davis2, B.W. Filippone5, M. Gericke6, R. Golub4, K. Hatanaka7, M. Hayden8, T.M. Ito9, S. Jeong10, I. Kato2, S. Komamiya11, E. Korobkina4, E. Korkmaz12, L. Lee6, Y. Masuda9, K. Matsuta13, A. Micherdzinska1, W.D. Ramsay6, S.A. Page6, B. Plaster14, I. Tanihata7, W.T.H. van Oers6, Y. Watanabe10, S. Yamashita11, and T. Yoshioka11 1University of Winnipeg, Winnipeg, MB 2TRIUMF, Vancouver, BC 3Oak Ridge National Laboratory, Oak Ridge, TN, USA 4North Carolina State University, Raleigh, NC, USA 5California Institute of Technology, Pasadena, CA, USA 6University of Manitoba, Winnipeg, MB 7Research Center for Nuclear Physics, Osaka University, Osaka, Japan 8Simon Fraser University, Burnaby, BC 9Los Alamos National Laboratory, Los Alamos, NM, USA 10KEK, 1-1 Oho, Tsukuba, Ibaraki, Japan 11The University of Tokyo, Tokyo, Japan 12University of Northern British Columbia, Prince George, BC 13Osaka University, Toyonaka, Osaka, Japan 14University of Kentucky, Lexington, KY, USA March 17, 2008 1 Executive Summary We propose the construction of the world’s highest density source of ultracold neutrons (UCN) at TRIUMF. The truly high density that could be obtained at TRIUMF would allow a class of precision measurements to be conducted with significantly improved statistical and systematic uncertainties, and thus more significant results. This source would therefore make a major impact on studies of fundamental physics with UCN that would complement and enhance the ISAC program. A window of opportunity exists to capitalize on the successes of Y. Masuda’s group at KEK and at RCNP, thereby allowing the TRIUMF project to surpass other proposed sources elsewhere. -
Letters to the Editors
NUCLEAR SCIENCE AND ENGINEERING: 10, 362-366 (1961) Letters to the Editors Spatial Dependence of Thermal-Neutron cantly alter the experimental determination of the thermal utilization. Spectra and the Interpretation of Thermal Second, the pronounced spatial dependence of the Utilization Measurements thermal-neutron spectra will not usually permit equating the activation ratio to the flux ratio. Bigham has measured the temperature dependence of the ratio of the Pu239 to The thermal utilization, /, is usually defined as the U235 average fission cross sections in a Maxwellian spectrum number of thermal neutrons absorbed in the fuel per thermal (3). In light w^ater, at 50°C, a value of 1.44 was measured, 2b5 neutron absorbed in the lattice. For a low U enrichment whereas the value at 95°C was 1.51. Klein has made room lattice consisting of uranium fuel rods temperature measurements of Pu239 fission reaction rates relative to U235 in light-water uranium-metal lattices f <f>mVmNm*m(Tm) + 0cl Vol N0i (Tcl^cl)]"1 containing 1.3% U235 enrichment (4). The measured ratios ^ 1 0uFu[iV26 *26(ru) + iV28^28(Tu)] J of the Pu239 to U235 fission reaction rates below the cadmium where V is the volume of the material; N is the atomic cutoff is 2.00 for a 2.35:1 water-to-metal lattice, and 2.37 density; a (T) is the microscopic absorption cross section for a 1:1 water-to-metal lattice. These latter ratios are averaged over an appropriate thermal-neutron spectrum approximately proportional to the ratio of the average characterized by a neutron temperature, T; and <f> denotes Pu239 to U235 thermal-fission cross sections. -
JAERI-Review 2000-031
JAERI-Review 2000-031 Japan Atomic Energy Research Institute A-p»PnifYt>-fr{i, n^uf-timn^m%mm^m%mmM (T319-1195 f- (=f 319-1195 This report is issued irregularly. Inquiries about availability of the reports should be addressed to Research Information Division, Department of Intellectual Resources, Japan Atomic Energy Research Institute, Tokai-mura, Naka-gun, Ibaraki-ken T 319-1195, Japan. CO Japan Atomic Energy Research Institute, 2001 JAERI-Review 2000-031 £22 ** (2000^ 10 E 23 -^) T. Oft. Hft lc ICANS •. T319-H95 £ttfta&nvxfttta£&tii 2-4 JAERI-Review 2000-031 Outline of Spallation Neutron Source Engineering Noboru WATANABE* Center for Neutron Science Tokai Research Establishment Japan Atomic Energy Research Institute Tokai-mura, Naka-gun, Ibaraki-ken (Received October 23, 2000) Slow neutrons such as cold and thermal neutrons are unique probes which can determine structures and dynamics of condensed matter in atomic scale. The neutron scattering technique is indispensable not only for basic sciences such as condensed matter research and life science, but also for basic industrial technology in 21 century. It is believed that to survive in the science- technology competition in 21 century would be almost impossible without neutron scattering. However, the intensity of neutrons presently available is much lower than synchrotron radiation sources, etc. Thus, R&D of intense neutron sources become most important. The High-Intensity Proton Accelerator Project is now being promoted jointly by Japan Atomic Energy Research Institute and High Energy Accelerator Research Organization, but there has so far been no good text which covers all the aspects of pulsed spallation neutron sources. -
Neutron Activation Analysis
International Atomic Energy Agency INDC(NDS)-411 Distr.: G+PG I N DC INTERNATIONAL NUCLEAR DATA COMMITTEE Development of a Database for Prompt y-ray Neutron Activation Analysis Summary Report of the First Research Coordination Meeting IAEA Headquarters, Vienna, Austria 2 to 4 November 1999 Prepared by R. Paviotti-Corcuera and R.M. Lindstrom* IAEA Nuclear Data Section Vienna, Austria * Analytical Chemistry Division, NIST, Gaithersburg, Maryland, USA February 2000 IAEA NUCLEAR DATA SECTION, WAGRAMERSTRASSE 5. A-14OO VIENNA 31-12 f> Reproduced by the IAEA in Austria February 2000 INDC(NDS)-411 Distr.: G+PG Development of a Database for Prompt y- Neutron Activation Analysis Summary Report of the First Research Coordination Meeting IAEA Headquarters, Vienna, Austria 2 to 4 November 1999 Prepared by R. Paviotti-Corcuera and R.M. Lindstrom* IAEA Nuclear Data Section Vienna, Austria * Analytical Chemistry Division, National Institute of Standards and Technology, Gaithersburg, Maryland, USA Abstract This report summarizes the presentations, recommendations and conclusions of the First Research Co-ordination Meeting on Development of a Database for Prompt y-ray Neutron Activation Analysis. Neutron-capture Prompt y-ray Activation Analysis (PGAA) is a non-destructive radioanalytical method, capable of rapid or in-situ simultaneous multielement analysis of many elements of the Periodic Table, from hydrogen to uranium, in the same sample. Inaccuracy and incompleteness of the data available for use in PGAA are a significant handicap in the qualitative and quantitative analysis of complicated capture-gamma spectra. The goal of this CRP is to replace the twenty-year-old data from a single laboratory with something fundamentally new: an evaluated database which includes a combination of evaluated nuclear physics data, physical theory, and recent measurements. -
Thermal Neutron Capture Cross Sections Resonance Integrals and G-Factors
International Atomic Energy Agency INDC(NDS)-440 Distr. PG+R I N D C INTERNATIONAL NUCLEAR DATA COMMITTEE THERMAL NEUTRON CAPTURE CROSS SECTIONS RESONANCE INTEGRALS AND G-FACTORS S.F. Mughabghab Brookhaven National Laboratory Upton, NY 11973-5000 U.S.A. Research carried out under the auspices of the U.S. Department of Energy under Prime Contract No. DE-AC02-98CH10886, and under IAEA contract No 11376/USA February 2003 IAEA NUCLEAR DATA SECTION, WAGRAMER STRASSE 5, A-1400 VIENNA Reproduced by the IAEA in Austria February 2003 INDC(NDS)-440 Distr. PG+R THERMAL NEUTRON CAPTURE CROSS SECTIONS RESONANCE INTEGRALS AND G-FACTORS S.F. Mughabghab Brookhaven National Laboratory Upton, NY 11973-5000 U.S.A. Research carried out under the auspices of the U.S. Department of Energy under Prime Contract No. DE-AC02-98CH10886, and under IAEA contract No 11376/USA Abstract The thermal radiative capture cross sections and resonance integrals of elements and isotopes with atomic numbers from 1 to 83 (as well as 232Th and 238U) have been re-evaluated by taking into consideration all known pertinent data published since 1979. This work has been undertaken as part of an IAEA co-ordinated research project on "Prompt capture gamma-ray activation analysis". Westcott g-factors for radiative capture cross sections at a temperature of 300K were computed by utilizing the INTER code and ENDF-B/VI (Release 8) library files. The temperature dependence of the Westcott g-factor is illustrated for 113Cd , 124Xe and 157Gd at temperatures of 150, 294 and 400K. Comparisons have also been made of the newly evaluated capture cross sections of 6Li, 7Li, 12C and 207Pb with those determined by the k0 method. -
AE-309 Measurement of the Decay of Thermal Neutrons in Water
AE-309 UDC 53S.12S.5.161 539.125.5.523 Measurement of the Decay of Thermal Neutrons in Water Poisoned with the Non-1/v Neutron Absorber Cadmium L. G. Larsson and E. Möller AKTIEBOLAGET ATOMENERGI STOCKHOLM, SWEDEN 1967 AE-309 •MEASUREMENT OF THE DECAY OF THERMAL NEUTRONS IN WATER POISONED WITH THE NON-l/v NEUTRON ABSORBER CADMIUM L G Larsson ' and E Möller SUMMARY Measurements have been made of the decay constant of thermal neutrons in water poisoned with the non-1/v absorber cadmium. An experimental method has been used in which proper spatial integration of the neutron flux enables data, representative of the infinite medium to be accumulated without waiting for the establishment of a fundamental mode distribution. The change in effective cross section with concen- daeff tration of the dissolved cadmium, —rr=—. has been determined for in- dN finite medium at 20 C. Two- and three parameter fits of the decay- constant yield -(0. 32 ± 0. 09) • 1 O-1 7 barn cm3 and -(0. 47 ± 0. 1 0) • 1 0"1 7 3 barn cm , respectively. Earlier published measurements have resulted in two to five times larger values, whereas a published calculated value -17 3 for Nelkin's model is - 0. 33 • 10 barn cm . *) Now at Research Institute of Swedish National Defence. Printed and distributed in January 1968. LIST OF CONTENTS Page 1 . Introduction 2. Principles of the experiment 3. Results of the measurements 4. Discussion of the results 5. Conclusion Acknowledgements References Table I Table II Figures - 3 - 1 . INTRODUCTION Various methods may be used for integral studies of thermal neu tron scattering in moderators. -
Neutron Physics
Neutron Physics MIT Department of Physics (Dated: October 16, 2014) The technique of time-of-flight spectroscopy with a mechanical beam chopper is used to study the properties of thermal neutrons in a beam emerging from the MIT Research Reactor (MITR-II) at the MIT Nuclear Reactor Laboratory. First, the distribution in velocity of the neutrons is measured, and the results are compared with the Maxwell-Boltzmann distribution for the temperature of the reactor. Second, the De Broglie relation between wavelength and momentum of neutrons is determined from measurements of the angle of Bragg reflection of the beam from a copper crystal as a function of the velocity measured by time-of-flight. Third, the absorption cross sections of several elements are measured, and the 1=v dependence of the boron cross section is observed. PREPARATORY QUESTIONS the experiment remotely from Junior Lab. Any time you need to visit the experiment in the reactor containment Please visit the Neutron Physics chapter on the 8.13x building, you must be escorted by an authorized Junior website at mitx.mit.edu to review the background ma- Lab staff member or NRL personnel. NRL protocols terial for this experiment. Answer all questions found in require that you wear long pants and closed-toe the chapter. Work out the solutions in your laboratory shoes without pointed heels whenever visiting the notebook; submit your answers on the web site. facility. For the purpose of Junior Laboratory experimentation, the following steps should be taken in order to have access SAFETY to the reactor: 1. First, complete the trainings described in the Neu- Training Requirements tron Spectrometer Operation Manual. -
MODELING and DESIGN of a SILICON-BASED HIGH-PRESSURE 3HE REPLACEMENT NEUTRON DETECTOR a THESIS in Physics Presented to the Fa
MODELING AND DESIGN OF A SILICON-BASED HIGH-PRESSURE 3HE REPLACEMENT NEUTRON DETECTOR A THESIS IN Physics Presented to the Faculty of the University of Missouri-Kansas City in partial fulfillment of the requirements for the degree MASTER OF PHYSICS by BRENT JOYNER ROGERS B.A. Business Administration, Park University, 2007 Kansas City, Missouri 2015 © 2015 BRENT JOYNER ROGERS ALL RIGHTS RESERVED MODELING AND DESIGN OF A SILICON-BASED HIGH-PRESSURE 3HE REPLACEMENT NEUTRON DETECTOR Brent Joyner Rogers, Candidate for the Master of Science Degree University of Missouri-Kansas City, 2015 ABSTRACT 3He has stood as a major isotope used for neutron detection for many years. Due to national concerns of a possible 3He shortage, significant effort has been put forth, in the form of 3He alternative research, to push the community to develop more cost effective and higher intrinsic efficiency devices. The major focus of this thesis is on how a Si-based micro- structured neutron detector (MSND) assembly, of size and shape comparable to that of a commercially available high-pressure 3He-based neutron detector, can yield higher neutron detection efficiencies than its 3He-based counterpart. With careful consideration of MSND assembly geometry, along with clever utilization of neutron moderating high-density polyethylene (HDPE), a high-pressure 3He replacement (HP-HeRep) device, consisting of eighty tightly packed 1-cm2 MSND’s, has been designed and will be compared with a high- performance 8.3 absolute atmosphere 3He-based neutron detector. The MSND, designed and developed by the S.M.A.R.T. Lab at Kansas State University, is a high purity Si-based, highly anisotropic pin structure diode (i.e., 350-μm by 20-μm trenches in a 525-μm thick substrate).