Resilience Vs. Decline
Total Page:16
File Type:pdf, Size:1020Kb
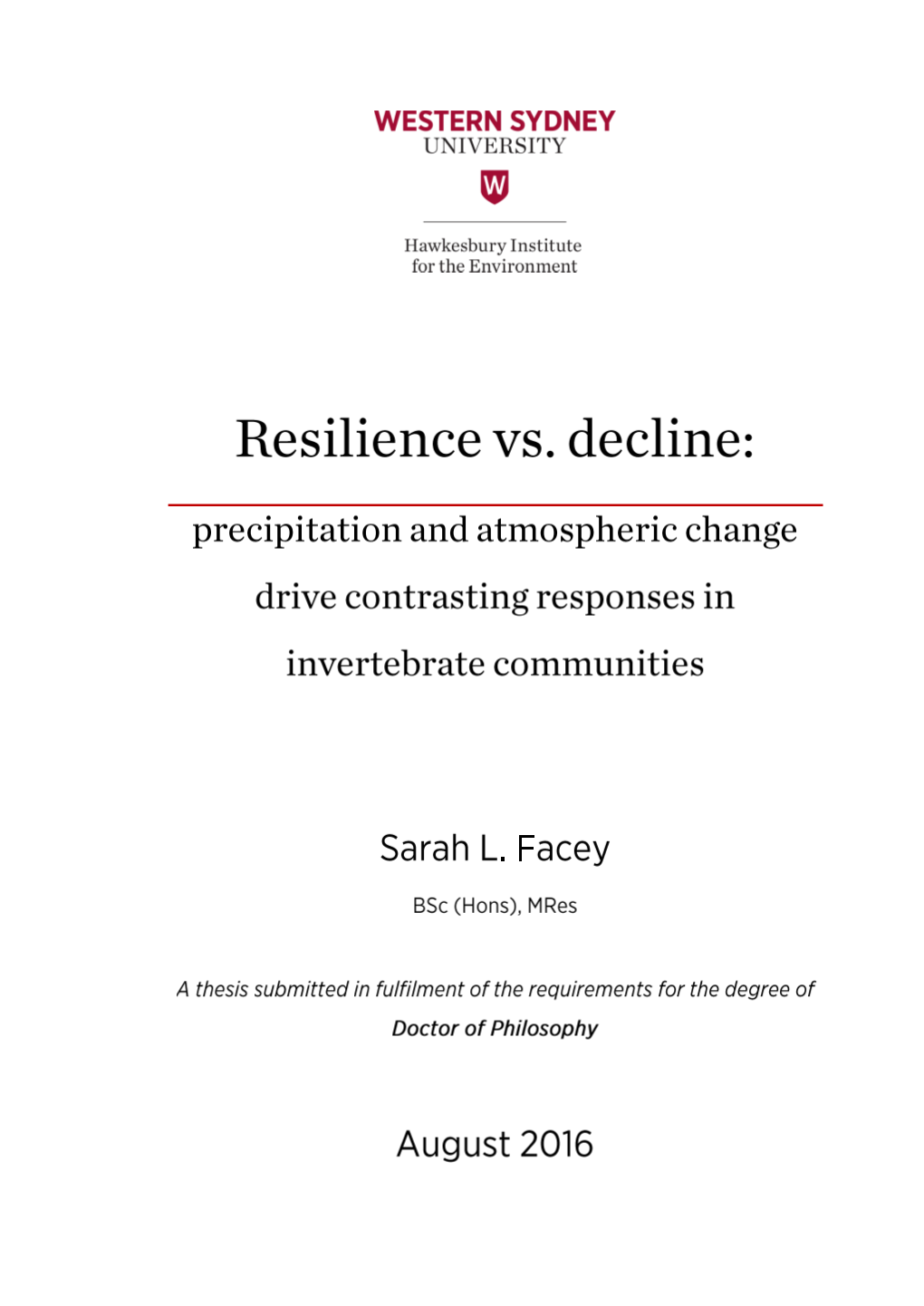
Load more
Recommended publications
-
Assessing the Presence and Distribution of 23 Hawaiian Yellow-Faced Bee Species on Lands Adjacent to Military Installations on O‘Ahu and Hawai‘I Island
The Hawai`i-Pacific Islands Cooperative Ecosystems Studies Unit & Pacific Cooperative Studies Unit UNIVERSITY OF HAWAI`I AT MĀNOA Dr. David C. Duffy, Unit Leader Department of Botany 3190 Maile Way, St. John #408 Honolulu, Hawai’i 96822 Technical Report 185 Assessing the presence and distribution of 23 Hawaiian yellow-faced bee species on lands adjacent to military installations on O‘ahu and Hawai‘i Island September 2013 Karl N. Magnacca1 and Cynthia B. K. King 2 1 Pacific Cooperative Studies Unit, University of Hawai‘i at Mānoa, Department of Botany, 3190 Maile Way Honolulu, Hawai‘i 96822 2 Hawaii Division of Forestry & Wildlife Native Invertebrate Program 1151 Punchbowl Street, Room 325 Honolulu, Hawaii 96813 PCSU is a cooperative program between the University of Hawai`i and U.S. National Park Service, Cooperative Ecological Studies Unit. Author Contact Information: Karl N. Magnacca. Phone: 808-554-5637 Email: [email protected] Hawaii Division of Forestry & Wildlife Native Invertebrate Program 1151 Punchbowl Street, Room 325 Honolulu, Hawaii 96813. Recommended Citation: Magnacca, K.N. and C.B.K. King. 2013. Assessing the presence and distribution of 23 Hawaiian yellow- faced bee species on lands adjacent to military installations on O‘ahu and Hawai‘i Island. Technical Report No. 185. Pacific Cooperative Studies Unit, University of Hawai‘i, Honolulu, Hawai‘i. 39 pp. Key words: Hylaeus, Colletidae, Apoidea, Hymenoptera, bees, insect conservation Place key words: Oahu, Schofield Barracks, Hawaii, Puu Waawaa, Mauna Kea, Pohakuloa, North Kona Editor: David C. Duffy, PCSU Unit Leader (Email: [email protected]) Series Editor: Clifford W. Morden, PCSU Deputy Director (Email: [email protected]) About this technical report series: This technical report series began in 1973 with the formation of the Cooperative National Park Resources Studies Unit at the University of Hawai'i at Mānoa. -
Highlights Section Reports
DACS-P-00124 Volume 54, Number 5, September - October 2015 DPI’s Bureau of Entomology, Nematology and Plant Pathology (the botany section is included in this bureau) produces TRI- OLOGY six times a year, covering two months of activity in each issue. The report includes detection activities from nursery plant inspections, routine and emergency program surveys, and requests for identification of plants and pests from the public. Samples are also occasionally sent from other states or countries for identification or diagnosis. Highlights Following are a few of the notable entries from this Section Reports volume of TRI-OLOGY. These entries are reports of interesting plants or unusual pests, some of Botany 2 which may be problematic. See Section Reports for complete information. Entomology 6 Bactrocera dorsalis, Oriental fruit fly, Bactrocera dorsalis, Oriental fruit fly. Based on female Nematology 10 Photograph courtesy of Gary J. Steck, the large number of flies detected in a concentrated DPI area of the Redland Agricultural District in late Plant Pathology 12 August, a quarantine area regulating the movement of oriental fruit fly host plants was established on 4 September 2015. All entities within the quarantine area of 98 square miles that are involved with the production, sale or distribution of oriental fruit fly host material have been placed under a compliance agreement outlining operational procedures and Pseudocercospora artanthes typical program requirements. irregular leaf spots caused by the fungal pathogen on Piper auritum (Vera Cruz Pseudocercospora artanthes (leaf spot) was found pepper). infecting Piper auritum (Vera Cruz pepper) at the Photograph courtesy of Robert M. Leahy, USDA Jacksonville Zoo and Gardens in Duval County. -
Black House Ants (Ochetellus Glaber), Summary Inset Showing a Queen Ant Black House Ants Are Native to Australia and Are a Common House-Infesting Ant Species
July 2018 Factsheet Black house ants (Ochetellus glaber) Ants to watch out for Red imported fire ants, yellow crazy ants, electric ants and carpenter ants, all pose a serious social, economic and environmental threat to Western Australia. If you suspect you have these ants or any ants you haven’t seen before, please contact us on freecall 1800 084 881. Black house ants (Ochetellus glaber), Summary inset showing a queen ant Black house ants are native to Australia and are a common house-infesting ant species. These ants do not bite or sting and are active day and night. Where are they found? In the home they are commonly attracted to sweet liquids and foods and are often drawn to the kitchen, laundry and bathroom. They naturally nest and forage in trees, feeding on insects, honeydew and nectar. Damage These ants rarely cause direct damage but are considered household pests as they are one of the few ant species that will nest inside. They can be found nesting in areas such as roof and wall spaces, rolled up awnings, pot plants, and in-between flat packed items. They may also Contact infest electrical items such as kettles, microwave ovens, computers or clock radios. Pest and Disease Information Service (PaDIS) Treatment Call: (08) 9368 3080 Control of these ants when numbers are low is advisable. When numbers are high and multiple nests have been Email: [email protected] established, control can be increasingly difficult. If nests are exposed they can be sprayed with fly spray, otherwise baits containing borates (borax) are effective for this sweet- feeding ant species. -
Hymenoptera: Formicidae
16 The Weta 30: 16-18 (2005) Changes to the classification of ants (Hymenoptera: Formicidae) Darren F. Ward School of Biological Sciences, Tamaki Campus, Auckland University, Private Bag 92019, Auckland ([email protected]) Introduction This short note aims to update the reader on changes to the subfamily classification of ants (Hymenoptera: Formicidae). Although the New Zealand ant fauna is very small, these changes affect the classification and phylogeny of both endemic and exotic ant species in New Zealand. Bolton (2003) has recently proposed a new subfamily classification for ants. Two new subfamilies have been created, a revised status for one, and new status for four. Worldwide, there are now 21 extant subfamilies of ants. The endemic fauna of New Zealand is now classified into six subfamilies (Table 1), as a result of three subfamilies, Amblyoponinae, Heteroponerinae and Proceratiinae, being split from the traditional subfamily Ponerinae. Bolton’s (2003) classification also affects several exotic species in New Zealand. Three species have been transferred from Ponerinae: Amblyopone australis to Amblyoponinae, and Rhytidoponera chalybaea and R. metallica to Ectatomminae. Currently there are 28 exotic species in New Zealand (Table 1). Eighteen species have most likely come from Australia, where they are native. Eight are global tramp species, commonly transported by human activities, and two species are of African origin. Nineteen of the currently established exotic species are recorded for the first time in New Zealand as occurring outside their native range. This may result in difficulty in obtaining species-specific biological knowledge and assessing their likelihood of becoming successful invaders. In addition to the work by Bolton (2003), Phil Ward and colleagues at UC Davis have started to resolve the phylogenetic relationships among subfamilies and genera of all ants using molecular data (Ward et al, 2005). -
The Butterflies of Taita Hills
FLUTTERING BEAUTY WITH BENEFITS THE BUTTERFLIES OF TAITA HILLS A FIELD GUIDE Esther N. Kioko, Alex M. Musyoki, Augustine E. Luanga, Oliver C. Genga & Duncan K. Mwinzi FLUTTERING BEAUTY WITH BENEFITS: THE BUTTERFLIES OF TAITA HILLS A FIELD GUIDE TO THE BUTTERFLIES OF TAITA HILLS Esther N. Kioko, Alex M. Musyoki, Augustine E. Luanga, Oliver C. Genga & Duncan K. Mwinzi Supported by the National Museums of Kenya and the JRS Biodiversity Foundation ii FLUTTERING BEAUTY WITH BENEFITS: THE BUTTERFLIES OF TAITA HILLS Dedication In fond memory of Prof. Thomas R. Odhiambo and Torben B. Larsen Prof. T. R. Odhiambo’s contribution to insect studies in Africa laid a concrete footing for many of today’s and future entomologists. Torben Larsen’s contribution to the study of butterflies in Kenya and their natural history laid a firm foundation for the current and future butterfly researchers, enthusiasts and rearers. National Museums of Kenya’s mission is to collect, preserve, study, document and present Kenya’s past and present cultural and natural heritage. This is for the purposes of enhancing knowledge, appreciation, respect and sustainable utilization of these resources for the benefit of Kenya and the world, for now and posterity. Copyright © 2021 National Museums of Kenya. Citation Kioko, E. N., Musyoki, A. M., Luanga, A. E., Genga, O. C. & Mwinzi, D. K. (2021). Fluttering beauty with benefits: The butterflies of Taita Hills. A field guide. National Museums of Kenya, Nairobi, Kenya. ISBN 9966-955-38-0 iii FLUTTERING BEAUTY WITH BENEFITS: THE BUTTERFLIES OF TAITA HILLS FOREWORD The Taita Hills are particularly diverse but equally endangered. -
Surveying for Terrestrial Arthropods (Insects and Relatives) Occurring Within the Kahului Airport Environs, Maui, Hawai‘I: Synthesis Report
Surveying for Terrestrial Arthropods (Insects and Relatives) Occurring within the Kahului Airport Environs, Maui, Hawai‘i: Synthesis Report Prepared by Francis G. Howarth, David J. Preston, and Richard Pyle Honolulu, Hawaii January 2012 Surveying for Terrestrial Arthropods (Insects and Relatives) Occurring within the Kahului Airport Environs, Maui, Hawai‘i: Synthesis Report Francis G. Howarth, David J. Preston, and Richard Pyle Hawaii Biological Survey Bishop Museum Honolulu, Hawai‘i 96817 USA Prepared for EKNA Services Inc. 615 Pi‘ikoi Street, Suite 300 Honolulu, Hawai‘i 96814 and State of Hawaii, Department of Transportation, Airports Division Bishop Museum Technical Report 58 Honolulu, Hawaii January 2012 Bishop Museum Press 1525 Bernice Street Honolulu, Hawai‘i Copyright 2012 Bishop Museum All Rights Reserved Printed in the United States of America ISSN 1085-455X Contribution No. 2012 001 to the Hawaii Biological Survey COVER Adult male Hawaiian long-horned wood-borer, Plagithmysus kahului, on its host plant Chenopodium oahuense. This species is endemic to lowland Maui and was discovered during the arthropod surveys. Photograph by Forest and Kim Starr, Makawao, Maui. Used with permission. Hawaii Biological Report on Monitoring Arthropods within Kahului Airport Environs, Synthesis TABLE OF CONTENTS Table of Contents …………….......................................................……………...........……………..…..….i. Executive Summary …….....................................................…………………...........……………..…..….1 Introduction ..................................................................………………………...........……………..…..….4 -
Black House Ant (Black Household Ant)
Pest Profile Photo credit: April Nobie, California Academy of Sciences (Specimen CASENT 0003317; fromhttps://www.antweb.org) Common Name: Black house ant (black household ant) Scientific Name: Ochetellus glauber Order and Family: Order Hymenoptera; Family Formicidae Size and Appearance: Length (mm) Appearance Egg Larva/Nymph Adult Workers are 2 - 3 mm Workers are brown to black in color with a one-part waist, 12-segmented antennae, and the abdomen has Queens are 5.2 - 5.5 a pore at its end (acidiopore). These ants do not have mm a stinger. Males are 1.6 mm Pupa (if applicable) Type of feeder (Chewing, sucking, etc.): Chewing Host(s): These black house ant is omnivorous feeding on insects, floral nectaries, and the honeydew of hemipteran plant pest. Description of Damage (larvae and adults): The black house ant is a nuisance pest that will nest in buildings, such as homes, where it forages on human foods, especially sweets. They often nest and forage in kitchens and bathrooms but also nest in walls, potted plants, rolled up awnings, and other small spaces. They may also be attracted to electrical wiring. Though the ants lack a stinger, they do bite. The black house ant is considered a threat to ecosystems and may disrupt the biological control of some agricultural pests. The black house ant is an introduced species from Australia and is found in Florida and Hawaii. References: Black house ant. (2018). Factsheet, Western Australia Agricultural Authority. Retrieved from https://www.agric.wa.gov.au/sites/gateway/files/Pest%20and%20Disease%20Information%20S ervice%20%28PaDIS%29%20-%20Ant%20factsheet%20- %20Black%20house%20ants%20%28A362015%29%20v2.pdf Ochetellus glauber. -
Johnston Atoll Species List Ryan Rash
Johnston Atoll Species List Ryan Rash Birds X: indicates species that was observed but not Anatidae photographed Green-winged Teal (Anas crecca) (DOR) Northern Pintail (Anas acuta) X Kingdom Ardeidae Cattle Egret (Bubulcus ibis) Phylum Charadriidae Class Pacific Golden-Plover (Pluvialis fulva) Order Fregatidae Family Great Frigatebird (Fregata minor) Genus species Laridae Black Noddy (Anous minutus) Brown Noddy (Anous stolidus) Grey-Backed Tern (Onychoprion lunatus) Sooty Tern (Onychoprion fuscatus) White (Fairy) Tern (Gygis alba) Phaethontidae Red-Tailed Tropicbird (Phaethon rubricauda) White-Tailed Tropicbird (Phaethon lepturus) Procellariidae Wedge-Tailed Shearwater (Puffinus pacificus) Scolopacidae Bristle-Thighed Curlew (Numenius tahitiensis) Ruddy Turnstone (Arenaria interpres) Sanderling (Calidris alba) Wandering Tattler (Heteroscelus incanus) Strigidae Hawaiian Short-Eared Owl (Asio flammeus sandwichensis) Sulidae Brown Booby (Sula leucogaster) Masked Booby (Sula dactylatra) Red-Footed Booby (Sula sula) Fish Acanthuridae Achilles Tang (Acanthurus achilles) Achilles Tang x Goldrim Surgeonfish Hybrid (Acanthurus achilles x A. nigricans) Black Surgeonfish (Ctenochaetus hawaiiensis) Blueline Surgeonfish (Acanthurus nigroris) Convict Tang (Acanthurus triostegus) Goldrim Surgeonfish (Acanthurus nigricans) Gold-Ring Surgeonfish (Ctenochaetus strigosus) Orangeband Surgeonfish (Acanthurus olivaceus) Orangespine Unicornfish (Naso lituratus) Ringtail Surgeonfish (Acanthurus blochii) Sailfin Tang (Zebrasoma veliferum) Yellow Tang (Zebrasoma flavescens) -
Invasive Ants Reduce Nesting Success of an Endangered Hawaiian Yellow-Faced Bee, Hylaeus Anthracinus
NeoBiota 64: 137–154 (2021) A peer-reviewed open-access journal doi: 10.3897/neobiota.64.58670 RESEARCH ARTICLE NeoBiota https://neobiota.pensoft.net Advancing research on alien species and biological invasions Invasive ants reduce nesting success of an endangered Hawaiian yellow-faced bee, Hylaeus anthracinus Sheldon Plentovich1, Jason R. Graham2, William P. Haines3, Cynthia B.A. King3 1 Pacific Islands Coastal Program, U.S. Fish and Wildlife Service, 300 Ala Moana Blvd, Rm 3-122, Honolulu, HI 96750, USA 2 Bishop Museum, 1525 Bernice Street, Honolulu, HI 96817, USA 3 Hawai‘i Department of Land and Natural Resources, Division of Forestry and Wildlife, 1151 Punchbowl St. Rm. 325, Honolulu, HI 96813, USA Corresponding author: Sheldon Plentovich ([email protected]) Academic editor: J. Sun | Received 23 September 2020 | Accepted 21 December 2020 | Published 28 January 2021 Citation: Plentovich S, Graham JR, Haines WP, King CBA (2021) Invasive ants reduce nesting success of an endangered Hawaiian yellow-faced bee, Hylaeus anthracinus. NeoBiota 64: 137–154. https://doi.org/10.3897/neobiota.64.58670 Abstract Hawaii has a single group of native bees belonging to the genus Hylaeus (Hymenoptera: Colletidae) and known collectively as Hawaiian yellow-faced bees. The majority of the 63 species have experienced sig- nificant declines in range and population. In 2016, seven species received federal protection under the Endangered Species Act of 1973. Competitors and predators, such as invasive bees, wasps and ants, are thought to be important drivers of range reductions and population declines, especially at lower elevations where more non-native species occur. We evaluated the effects of invasive ants on nesting Hylaeus anthra- cinus using artificial nest blocks that allowed us to track nest construction and development. -
Coastal/ Marine Zones of Haleakalä
HöÿikeHöÿike oo HaleakaläHaleakalä Haleakalä revealed: an opening to view our past and embrace our future Reveal yourself, summit to sea A Multidisciplinary, Science-Based Environmental Education Curriculum for High Schools ••••• Produced by Hawaiÿi Natural History Association Nä Kumu o Haleakalä Haleakalä National Park The Nature Conservancy Major funding provided by the Strong Foundation Additional support provided by the Alexander & Baldwin Foundation, Atherton Family Foundation, Fred Baldwin Memorial Foundation, and Cooke Foundation, Limited Höÿike o Haleakalä A Multidisciplinary, Science-Based Environmental Education Curriculum for High Schools Produced by Hawaiÿi Natural History Association Nä Kumu o Haleakalä Haleakalä National Park The Nature Conservancy Major funding provided by the Strong Foundation Additional support provided by the Alexander & Baldwin Foundation, Atherton Family Foundation, Fred Baldwin Memorial Foundation, and Cooke Foundation, Limited © Copyright 2001 by Hawaiÿi Natural History Association Post Office Box 74 Hawaiÿi National Park, Hawaiÿi 96718 All rights reserved Cover art and border: Sophie Cayless Design and layout: Michele Archie and Howard Terry, The Harbinger Institute Höÿike o Haleakalä Development Team Elizabeth Anderson Project Coordinator, Haleakalä National Park Michele Archie Writer, The Harbinger Institute Sandy Buczynski Advisor, Seabury Hall Ann Coopersmith Advisor, Maui Community College Jackie Davis Advisor, Baldwin High School Ann Fielding Writer, researcher Carol Gentz Project Coordinator, The Nature Conservancy Keith Ideoka Advisor, Lahainaluna High School Lyle Kajihara Advisor, King Kekaulike High School Lloyd Loope Scientific Advisor, U.S. Geological Survey, Biological Resources Division, Haleakalä National Park Kim Martz Researcher and advisor, U.S. Geological Survey, Biological Resources Division, Haleakalä National Park Dan Schulte Advisor, St. Anthony High School Forest Starr Researcher and advisor, U.S. -
Download PDF File
Myrmecological News 20 Digital supplementary material Digital supplementary material to LANAN, M. 2014: Spatiotemporal resource distribution and foraging strategies of ants (Hymenoptera: Formicidae). – Myrmecological News 20: 53-70. Tab. S1: Data and citations for information presented in Figure 3 and Figure S1. Note that no data in any column indi- cates a lack of reports in the literature. For instance, no data in the column for nesting strategy should not be interpreted as a positive report of strict monodomy, and ants may collect foods that have not been reported. Species Resource collected Foraging strategy Nesting strategy Acanthognathus rudis Small prey: collembola Solitary foraging (GRONENBERG & al. 1998) (GRONENBERG & al. 1998) Acromyrmex ambiguus Leaves (FOWLER 1985, Trunk trails, partially subterranean (FOWLER SAVERSCHEK & ROCES 2011) 1985) trails (SAVERSCHEK & ROCES 2011) Acromyrmex balzani Grass (LOPES & al. 2004) Recruitment, type = ? (LOPES & al. 2004) Polydomy (ICHINOSE Solitary foraging (PODEROSO & al. 2009) & al. 2006) Solitary foraging (FOWLER 1985) Acromyrmex coronatus Leaves (WETTERER 1995) Trunk trails (WETTERER 1995) Acromyrmex crassispinus Leaves (FOWLER 1985) Two to five trunk trails (FOWLER 1985) Acromyrmex disciger Leaves (FOWLER 1985) Trunk trails (FOWLER 1985) Acromyrmex fracticornis Grass (FOWLER & ROBINSON 1977) Solitary (FOWLER & ROBINSON 1977) Acromyrmex heyeri Grass (BOLLAZZI & ROCES 2011) Trunk trails (FOWLER 1985, BOLLAZZI & ROCES 2011) Acromyrmex hispidus fallax Leaves (FOWLER 1985) Trunk trails (FOWLER 1985) Acromyrmex laticeps Leaves (FOWLER 1985) Trunk trails (FOWLER 1985) Acromyrmex lobicornis Leaves (ELIZALDE & FARJI-BERNER Trunk trails (ELIZALDE & FARJI-BERNER 2012) 2012) Acromyrmex lundii Leaves (FOWLER 1988), mush- Trunk trails (FOWLER 1988) rooms (LECHNER & JOSENS 2012) Acromyrmex niger Leaves (SOUSA-SOUTO & al. 2005) Trunk trails (SOUSA-SOUTO & al. -
Download Document
SANBI Biodiversity Series 16 Butterflies of South Africa’s National Botanical Gardens An illustrated checklist compiled by Christopher K. Willis & Steve E. Woodhall Pretoria 2010 SANBI Biodiversity Series The South African National Biodiversity Institute (SANBI) was established on 1 Sep- tember 2004 through the signing into force of the National Environmental Manage- ment: Biodiversity Act (NEMBA) No. 10 of 2004 by President Thabo Mbeki. The Act expands the mandate of the former National Botanical Institute to include responsibili- ties relating to the full diversity of South Africa’s fauna and flora, and builds on the internationally respected programmes in conservation, research, education and visitor services developed by the National Botanical Institute and its predecessors over the past century. The vision of SANBI: Biodiversity richness for all South Africans. SANBI’s mission is to champion the exploration, conservation, sustainable use, appre- ciation and enjoyment of South Africa’s exceptionally rich biodiversity for all people. SANBI Biodiversity Series publishes occasional reports on projects, technologies, work- shops, symposia and other activities initiated by or executed in partnership with SANBI. Photographs: Steve Woodhall, unless otherwise noted Technical editing: Emsie du Plessis Design & layout: Sandra Turck Cover design: Sandra Turck Cover photographs: Front: Pirate (Christopher Willis) Back, top: African Leaf Commodore (Christopher Willis) Back, centre: Dotted Blue (Steve Woodhall) Back, bottom: Green-veined Charaxes (Christopher Willis) Citing this publication WILLIS, C.K. & WOODHALL, S.E. (Compilers) 2010. Butterflies of South Africa’s National Botanical Gardens. SANBI Biodiversity Series 16. South African National Biodiversity Institute, Pretoria. ISBN 978-1-919976-57-0 © Published by: South African National Biodiversity Institute.