Basics of Molecular Biology
Total Page:16
File Type:pdf, Size:1020Kb
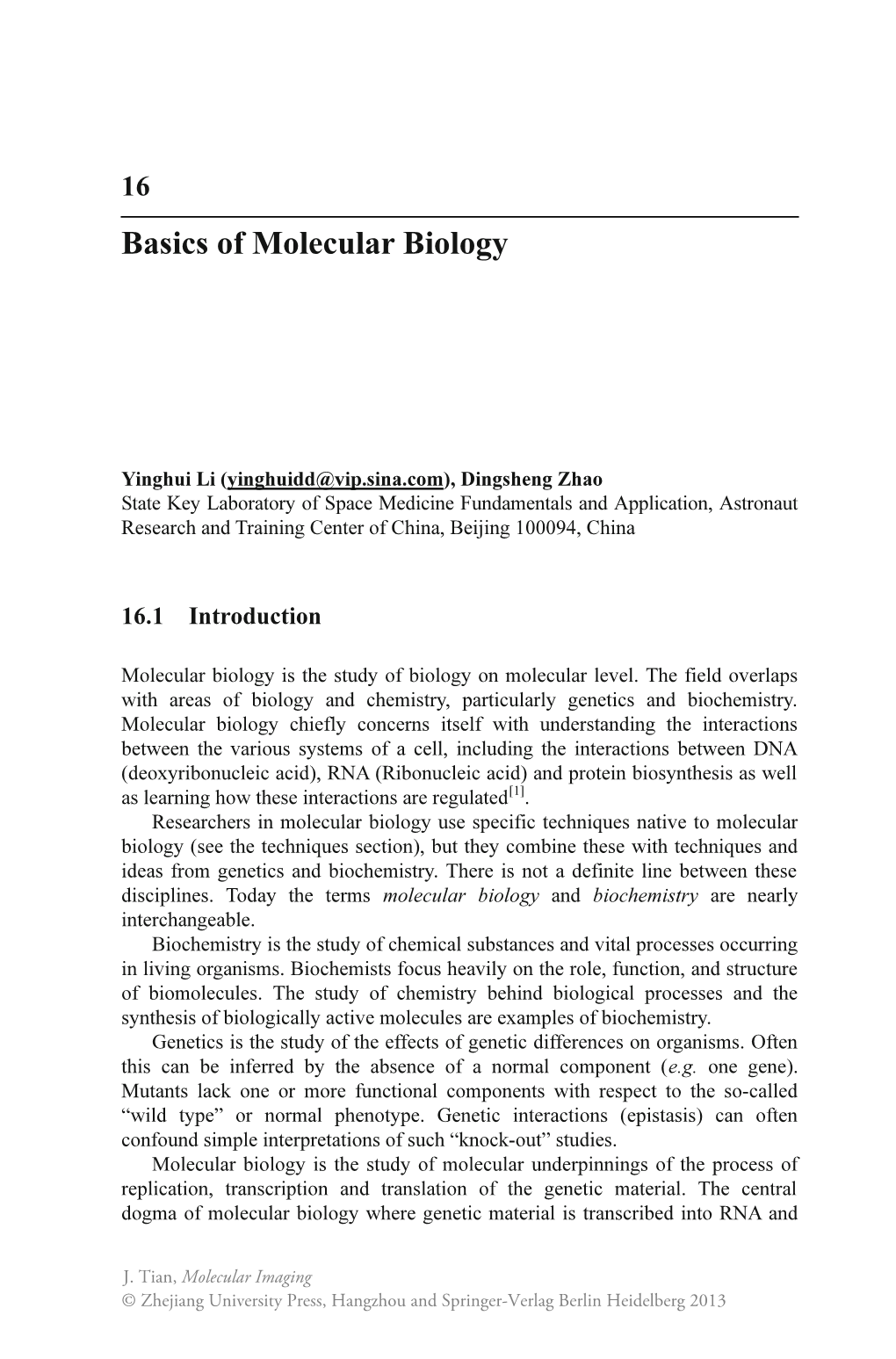
Load more
Recommended publications
-
The Functions of DNA Damage Factor RNF8 in the Pathogenesis And
Int. J. Biol. Sci. 2019, Vol. 15 909 Ivyspring International Publisher International Journal of Biological Sciences 2019; 15(5): 909-918. doi: 10.7150/ijbs.31972 Review The Functions of DNA Damage Factor RNF8 in the Pathogenesis and Progression of Cancer Tingting Zhou 1, Fei Yi 1, Zhuo Wang 1, Qiqiang Guo 1, Jingwei Liu 1, Ning Bai 1, Xiaoman Li 1, Xiang Dong 1, Ling Ren 2, Liu Cao 1, Xiaoyu Song 1 1. Institute of Translational Medicine, China Medical University; Key Laboratory of Medical Cell Biology, Ministry of Education; Liaoning Province Collaborative Innovation Center of Aging Related Disease Diagnosis and Treatment and Prevention, Shenyang, Liaoning Province, China 2. Department of Anus and Intestine Surgery, First Affiliated Hospital of China Medical University, Shenyang, Liaoning Province, China Corresponding authors: Xiaoyu Song, e-mail: [email protected] and Liu Cao, e-mail: [email protected]. Key Laboratory of Medical Cell Biology, Ministry of Education; Institute of Translational Medicine, China Medical University; Collaborative Innovation Center of Aging Related Disease Diagnosis and Treatment and Prevention, Shenyang, Liaoning Province, 110122, China. Tel: +86 24 31939636, Fax: +86 24 31939636. © Ivyspring International Publisher. This is an open access article distributed under the terms of the Creative Commons Attribution (CC BY-NC) license (https://creativecommons.org/licenses/by-nc/4.0/). See http://ivyspring.com/terms for full terms and conditions. Received: 2018.12.03; Accepted: 2019.02.08; Published: 2019.03.09 Abstract The really interesting new gene (RING) finger protein 8 (RNF8) is a central factor in DNA double strand break (DSB) signal transduction. -
Mediator of DNA Damage Checkpoint Protein 1 Facilitates V(D)J Recombination in Cells Lacking DNA Repair Factor XLF
biomolecules Article Mediator of DNA Damage Checkpoint Protein 1 Facilitates V(D)J Recombination in Cells Lacking DNA Repair Factor XLF Carole Beck 1,2, Sergio Castañeda-Zegarra 1,2 , Camilla Huse 1,2, Mengtan Xing 1,2 and Valentyn Oksenych 1,2,3,* 1 Department of Clinical and Molecular Medicine (IKOM), Norwegian University of Science and Technology (NTNU), 7491 Trondheim, Norway; [email protected] (C.B.); [email protected] (S.C.-Z.); [email protected] (C.H.); [email protected] (M.X.) 2 St. Olavs Hospital, Trondheim University Hospital, Clinic of Medicine, Postboks 3250 Sluppen, 7006 Trondheim, Norway 3 Department of Biosciences and Nutrition (BioNuT), Karolinska Institutet, 14183 Huddinge, Sweden * Correspondence: [email protected] Received: 27 November 2019; Accepted: 24 December 2019; Published: 30 December 2019 Abstract: DNA double-strand breaks (DSBs) trigger the Ataxia telangiectasia mutated (ATM)-dependent DNA damage response (DDR), which consists of histone H2AX, MDC1, RNF168, 53BP1, PTIP, RIF1, Rev7, and Shieldin. Early stages of B and T lymphocyte development are dependent on recombination activating gene (RAG)-induced DSBs that form the basis for further V(D)J recombination. Non-homologous end joining (NHEJ) pathway factors recognize, process, and ligate DSBs. Based on numerous loss-of-function studies, DDR factors were thought to be dispensable for the V(D)J recombination. In particular, mice lacking Mediator of DNA Damage Checkpoint Protein 1 (MDC1) possessed nearly wild-type levels of mature B and T lymphocytes in the spleen, thymus, and bone marrow. NHEJ factor XRCC4-like factor (XLF)/Cernunnos is functionally redundant with ATM, histone H2AX, and p53-binding protein 1 (53BP1) during the lymphocyte development in mice. -
Biochemistry and the Genomic Revolution 1.1
Dedication About the authors Preface Tools and Techniques Clinical Applications Molecular Evolution Supplements Supporting Biochemistry, Fifth Edition Acknowledgments I. The Molecular Design of Life 1. Prelude: Biochemistry and the Genomic Revolution 1.1. DNA Illustrates the Relation between Form and Function 1.2. Biochemical Unity Underlies Biological Diversity 1.3. Chemical Bonds in Biochemistry 1.4. Biochemistry and Human Biology Appendix: Depicting Molecular Structures 2. Biochemical Evolution 2.1. Key Organic Molecules Are Used by Living Systems 2.2. Evolution Requires Reproduction, Variation, and Selective Pressure 2.3. Energy Transformations Are Necessary to Sustain Living Systems 2.4. Cells Can Respond to Changes in Their Environments Summary Problems Selected Readings 3. Protein Structure and Function 3.1. Proteins Are Built from a Repertoire of 20 Amino Acids 3.2. Primary Structure: Amino Acids Are Linked by Peptide Bonds to Form Polypeptide Chains 3.3. Secondary Structure: Polypeptide Chains Can Fold Into Regular Structures Such as the Alpha Helix, the Beta Sheet, and Turns and Loops 3.4. Tertiary Structure: Water-Soluble Proteins Fold Into Compact Structures with Nonpolar Cores 3.5. Quaternary Structure: Polypeptide Chains Can Assemble Into Multisubunit Structures 3.6. The Amino Acid Sequence of a Protein Determines Its Three-Dimensional Structure Summary Appendix: Acid-Base Concepts Problems Selected Readings 4. Exploring Proteins 4.1. The Purification of Proteins Is an Essential First Step in Understanding Their Function 4.2. Amino Acid Sequences Can Be Determined by Automated Edman Degradation 4.3. Immunology Provides Important Techniques with Which to Investigate Proteins 4.4. Peptides Can Be Synthesized by Automated Solid-Phase Methods 4.5. -
Exploring the Structure of Long Non-Coding Rnas, J
IMF YJMBI-63988; No. of pages: 15; 4C: 3, 4, 7, 8, 10 1 2 Rise of the RNA Machines: Exploring the Structure of 3 Long Non-Coding RNAs 4 Irina V. Novikova, Scott P. Hennelly, Chang-Shung Tung and Karissa Y. Sanbonmatsu Q15 6 Los Alamos National Laboratory, Los Alamos, NM 87545, USA 7 Correspondence to Karissa Y. Sanbonmatsu: [email protected] 8 http://dx.doi.org/10.1016/j.jmb.2013.02.030 9 Edited by A. Pyle 1011 12 Abstract 13 Novel, profound and unexpected roles of long non-coding RNAs (lncRNAs) are emerging in critical aspects of 14 gene regulation. Thousands of lncRNAs have been recently discovered in a wide range of mammalian 15 systems, related to development, epigenetics, cancer, brain function and hereditary disease. The structural 16 biology of these lncRNAs presents a brave new RNA world, which may contain a diverse zoo of new 17 architectures and mechanisms. While structural studies of lncRNAs are in their infancy, we describe existing 18 structural data for lncRNAs, as well as crystallographic studies of other RNA machines and their implications 19 for lncRNAs. We also discuss the importance of dynamics in RNA machine mechanism. Determining 20 commonalities between lncRNA systems will help elucidate the evolution and mechanistic role of lncRNAs in 21 disease, creating a structural framework necessary to pursue lncRNA-based therapeutics. 22 © 2013 Published by Elsevier Ltd. 24 23 25 Introduction rather than the exception in the case of eukaryotic 50 organisms. 51 26 RNA is primarily known as an intermediary in gene LncRNAs are defined by the following: (i) lack of 52 11 27 expression between DNA and proteins. -
Upstream Sequences Other Than AAUAAA Are Required for Efficient Messenger RNA 3’-End Formation in Plants
The Plant Cell, Vol. 2, 1261-1272, December 1990 O 1990 American Society of Plant Physiologists Upstream Sequences Other than AAUAAA Are Required for Efficient Messenger RNA 3’-End Formation in Plants Bradley D. Mogen, Margaret H. MacDonald, Robert Graybosch,’ and Arthur G. Hunt2 Plant Physiology/Biochemistry/MolecularBiology Program, Department of Agronomy, University of Kentucky, Lexington, Kentucky 40546-009 1 We have characterized the upstream nucleotide sequences involved in mRNA 3’-end formation in the 3‘ regions of the cauliflower mosaic virus (CaMV) 19S/35S transcription unit and a pea gene encoding ribulose-l,5-bisphosphate carboxylase small subunit (rbcs). Sequences between 57 bases and 181 bases upstream from the CaMV polyade- nylation site were required for efficient polyadenylation at this site. In addition, an AAUAAA sequence located 13 bases to 18 bases upstream from this site was also important for efficient mRNA 3’-end formation. An element located between 60 bases and 137 bases upstream from the poly(A) addition sites in a pea rbcS gene was needed for functioning of these sites. The CaMV -181/-57 and rbcS -137/-60 elements were different in location and sequence composition from upstream sequences needed for polyadenylation in mammalian genes, but resembled the signals that direct mRNA 3’-end formation in yeast. However, the role of the AAUAAA motif in 3’-end formation in the CaMV 3’ region was reminiscent of mRNA polyadenylation in animals. We suggest that multiple elements are involved in mRNA 3‘-end formation in plants, and that interactions of different components of the plant polyadenyl- ation apparatus with their respective sequence elements and with each other are needed for efficient mRNA 3‘-end formation. -
The Grammar of Transcriptional Regulation
Hum Genet (2014) 133:701–711 DOI 10.1007/s00439-013-1413-1 REVIEW PAPER The grammar of transcriptional regulation Shira Weingarten-Gabbay · Eran Segal Received: 11 June 2013 / Accepted: 24 December 2013 / Published online: 5 January 2014 © Springer-Verlag Berlin Heidelberg 2014 Abstract Eukaryotes employ combinatorial strategies to regulatory motifs (Levine and Tjian 2003) and exploit motif generate a variety of expression patterns from a relatively geometry as another dimension of combinatorial power small set of regulatory DNA elements. As in any other lan- for regulating transcription. Understanding the fundamen- guage, deciphering the mapping between DNA and expres- tal principles governing transcriptional regulation could sion requires an understanding of the set of rules that govern allow us to predict expression from DNA sequence, with basic principles in transcriptional regulation, the functional far-reaching implications. Most notably, in many human elements involved, and the ways in which they combine to diseases, genetic changes occur in non-coding regions such orchestrate a transcriptional output. Here, we review the cur- as gene promoters and enhancers. However, without under- rent understanding of various grammatical rules, including standing the grammar of transcriptional regulation, we the effect on expression of the number of transcription factor cannot tell which sequence changes affect expression and binding sites, their location, orientation, affinity and activity; how. For example, even for a single binding site, we do not co-association with different factors; and intrinsic nucleo- know the quantitative effects on expression of its location, some organization. We review different methods that are orientation, and affinity; whether these effects are general, used to study the grammar of transcription regulation, high- factor-specific, and/or promoter-dependent; and how they light gaps in current understanding, and discuss how recent depend on the intrinsic nucleosome organization. -
Expression Cloning of a Human Dual-Specificity Phosphatase TOSHIO ISHIBASHI, DONALD P
Proc. Nati. Acad. Sci. USA Vol. 89, pp. 12170-12174, December 1992 Biochemistry Expression cloning of a human dual-specificity phosphatase TOSHIO ISHIBASHI, DONALD P. BOTTARO, ANDREW CHAN, TORU MIKI, AND STUART A. AARONSON* Laboratory of Cellular and Molecular Biology, National Cancer Institute, Bethesda, MD 20892 Communicated by Leon A. Heppel, September 24, 1992 ABSTRACT Using an expression cloning strategy, we iso- substrate specificity for both phosphotyrosine and phospho- lated a cDNA encoding a human protein-tyrosine-phosphatase. serine. Bacteria expressing the kinase domain of the keratinocyte growth factor receptor (bek/fibroblast growth factor receptor 2) were infected with a fibroblast cDNA library in a phanid MATERIALS AND METHODS prokaryotic expression vector and screened with a monoclonal Plamid Construction of bek Kiame. The EcoRI-Sal I anti-phosphotyrosine antibody. Among several clones showing fragment of the mouse keratinocyte growth factor (KGF) decreased anti-phosphotyrosine recognition, one displayed receptor cDNA (15), which includes the kinase and carboxyl- phosphatase activity toward the kinase in vitro. The 4.1- terminal domains (residues 361-707), was ligated to the kilobase cDNA encoded a deduced protein of 185 amino ds EcoRP-Sal I fragment ofthe expression vector pCEV-lacZ to with limited sequence similar to the vaccinia virus phospha- generate the plasmid pCEV-bek (Fig. 1A). pCEV-lacZ is a tase VH1. The puriffied recombinant protein dephosphorlated pUC-based plasmid expression vector that produces a 3-gal several activated growth factor receptors, as well as serine- fusion protein under the control of the lac promoter (see phosphorylated casein, in vitro. Both serine and tyrosine phos- below). -
Interplay Between Ompa and Rpon Regulates Flagellar Synthesis in Stenotrophomonas Maltophilia
microorganisms Article Interplay between OmpA and RpoN Regulates Flagellar Synthesis in Stenotrophomonas maltophilia Chun-Hsing Liao 1,2,†, Chia-Lun Chang 3,†, Hsin-Hui Huang 3, Yi-Tsung Lin 2,4, Li-Hua Li 5,6 and Tsuey-Ching Yang 3,* 1 Division of Infectious Disease, Far Eastern Memorial Hospital, New Taipei City 220, Taiwan; [email protected] 2 Department of Medicine, National Yang Ming Chiao Tung University, Taipei 112, Taiwan; [email protected] 3 Department of Biotechnology and Laboratory Science in Medicine, National Yang Ming Chiao Tung University, Taipei 112, Taiwan; [email protected] (C.-L.C.); [email protected] (H.-H.H.) 4 Division of Infectious Diseases, Department of Medicine, Taipei Veterans General Hospital, Taipei 112, Taiwan 5 Department of Pathology and Laboratory Medicine, Taipei Veterans General Hosiptal, Taipei 112, Taiwan; [email protected] 6 Ph.D. Program in Medical Biotechnology, Taipei Medical University, Taipei 110, Taiwan * Correspondence: [email protected] † Liao, C.-H. and Chang, C.-L. contributed equally to this work. Abstract: OmpA, which encodes outer membrane protein A (OmpA), is the most abundant transcript in Stenotrophomonas maltophilia based on transcriptome analyses. The functions of OmpA, including adhesion, biofilm formation, drug resistance, and immune response targets, have been reported in some microorganisms, but few functions are known in S. maltophilia. This study aimed to elucidate the relationship between OmpA and swimming motility in S. maltophilia. KJDOmpA, an ompA mutant, Citation: Liao, C.-H.; Chang, C.-L.; displayed compromised swimming and failure of conjugation-mediated plasmid transportation. The Huang, H.-H.; Lin, Y.-T.; Li, L.-H.; hierarchical organization of flagella synthesis genes in S. -
POST-TRANSCRIPTIONAL REGULATION of AFP and Igm GENES
University of Kentucky UKnowledge University of Kentucky Doctoral Dissertations Graduate School 2011 POST-TRANSCRIPTIONAL REGULATION OF AFP AND IgM GENES Lilia M. Turcios University of Kentucky, [email protected] Right click to open a feedback form in a new tab to let us know how this document benefits ou.y Recommended Citation Turcios, Lilia M., "POST-TRANSCRIPTIONAL REGULATION OF AFP AND IgM GENES" (2011). University of Kentucky Doctoral Dissertations. 210. https://uknowledge.uky.edu/gradschool_diss/210 This Dissertation is brought to you for free and open access by the Graduate School at UKnowledge. It has been accepted for inclusion in University of Kentucky Doctoral Dissertations by an authorized administrator of UKnowledge. For more information, please contact [email protected]. ABSTRACT OF DISSERTATION Lilia M. Turcios The Graduate School University of Kentucky 2011 POST-TRANSCRIPTIONAL REGULATION OF AFP AND IgM GENES ABSTRACT OF DISSERTATION A dissertation submitted in partial fulfillment of the requirements for the degree of Doctor of Philosophy in the College of Medicine at the University of Kentucky By Lilia M. Turcios Director: Dr. Martha Peterson Lexington, KY 2011 Copyright © Lilia M. Turcios 2011 ABSTRACT OF DISSERTATION POST-TRANSCRIPTIONAL REGULATION OF AFP AND IgM GENES Gene expression can be regulated at multiple steps once transcription is initiated. I have studied two different gene models, the α-Fetoprotein (AFP) and the immunoglobulin heavy chain (IgM) genes, to better understand post-transcriptional gene regulation mechanisms. The AFP gene is highly expressed during fetal liver development and dramatically repressed after birth. There is a mouse strain-specific difference between adult levels of AFP, with BALB/cJ mice expressing 10 to 20-fold higher levels compared to other mouse strains. -
US5266490.Pdf
||||||||||||I|| US005266490A United States Patent (19) 11 Patent Number: 5,266,490 Davis et al. 45 Date of Patent: Nov.30, 1993 (54) MAMMALIAN EXPRESSION VECTOR Maniatis et al. (1990), Molecular Cloning: A Laboratory Manual, vol. 2, pp. 16.3-16.81. 75 Inventors: Samuel Davis, New York; George D. Martson (1987), DNA Cloning: A Practical Approach, Yancopoulos, Briarcliff Manor, both vol. 3, Chap. 4, pp. 59-88. of N.Y. Mizushima et al. (1990), Nucl. Acids Res. 18 (17): 5322. 73) Assignee: Regeneron Pharmaceuticals, Inc., Sambrook et al. (1988), Focus 10(3): 41-48. Tarrytown, N.Y. Simmons et al. (1988), J. Immunol. 141 (8): 2797-2800. Spector (1985), Genet. Engineering 7: 199-234. (21) Appl. No.: 678,408 Stamenkovic et al. (1988), J. Exp. Med. 167: 1975-1980. (22 Filed: Mar. 28, 1991 Urlaub et al. (1980), Proc. Nat. Acad. Sci USA 77(7): 51) Int. Cl.5...................... C12N 15/79; C12N 15/12; 426-4220. C12N 15/18 Woeltje et al. (1990), J. Biol. Chem. 265 (18): 10720-10725. 52) U.S. C. ................................ 435/320.1; 536/23.4; Wysocki et al. (1978); Proc. Nat. Acad. Sci USA 75(6): 536/23.5; 536/24.1 2844-2848. 58) Field of Search ................. 435/320.1, 69.1, 172.3; Seed, B. (Oct. 1987), Nature, vol. 329, pp. 840-842. 536/27, 24.1, 23.4, 23.5 Yanisch-Perron et al. (1985), Gene, vol. 33, pp. (56) References Cited 103-119. PUBLICATIONS Seed et al. (May, 1987), Proc. Nat. Acad. Sci USA, vol. 84, pp. 3365-3369. -
Technical Glossary
WBVGL 6/28/03 12:00 AM Page 409 Technical Glossary abortive infection: Infection of a cell where there is no net increase in the production of infectious virus. abortive transformation: See transitory (transient or abortive) transformation. acid blob activator: A regulatory protein that acts in trans to alter gene expression and whose activity depends on a region of an amino acid sequence containing acidic or phosphorylated residues. acquired immune deficiency syndrome (AIDS): A disease characterized by loss of cell-mediated and humoral immunity as the result of infection with human immunodeficiency virus (HIV). acute infection: An infection marked by a sudden onset of detectable symptoms usually followed by complete or apparent recovery. adaptive immunity (acquired immunity): See immunity. adjuvant: Something added to a drug to increase the effectiveness of that drug. With respect to the immune system, an adjuvant increases the response of the system to a particular antigen. agnogene: A region of a genome that contains an open reading frame of unknown function; origi- nally used to describe a 67- to 71-amino acid product from the late region of SV40. AIDS: See acquired immune deficiency syndrome. aliquot: One of a number of replicate samples of known size. a-TIF: The alpha trans-inducing factor protein of HSV; a structural (virion) protein that functions as an acid blob transcriptional activator. Its specificity requires interaction with certain host cel- lular proteins (such as Oct1) that bind to immediate-early promoter enhancers. ambisense genome: An RNA genome that contains sequence information in both the positive and negative senses. The S genomic segment of the Arenaviridae and of certain genera of the Bunyaviridae have this characteristic. -
Unitary Structure of Palindromes in DNA
bioRxiv preprint doi: https://doi.org/10.1101/2021.07.21.453288; this version posted July 22, 2021. The copyright holder for this preprint (which was not certified by peer review) is the author/funder. All rights reserved. No reuse allowed without permission. Unitary Structure of Palindromes in DNA Mehmet Ali Tibatan1, ∗ and Mustafa Sarısaman2, y 1Department of Biotechnology, Istanbul University, 34134, Vezneciler, Istanbul, Turkey 2Department of Physics, Istanbul University, 34134, Vezneciler, Istanbul, Turkey We investigate the quantum behavior encountered in palindromes within DNA structure. In par- ticular, we reveal the unitary structure of usual palindromic sequences found in genomic DNAs of all living organisms, using the Schwinger’s approach. We clearly demonstrate the role played by palin- dromic configurations with special emphasis on physical symmetries, in particular subsymmetries of unitary structure. We unveil the prominence of unitary structure in palindromic sequences in the sense that vitally significant information endowed within DNA could be transformed unchangeably in the process of transcription. We introduce a new symmetry relation, namely purine-purine or pyrimidine-pyrimidine symmetries (p-symmetry) in addition to the already known symmetry rela- tion of purine-pyrimidine symmetries (pp-symmetry) given by Chargaff’s rule. Therefore, important vital functions of a living organisms are protected by means of these symmetric features. It is un- derstood that higher order palindromic sequences could be generated in terms of the basis of the highest prime numbers that make up the palindrome sequence number. We propose that violation of this unitary structure of palindromic sequences by means of our proposed symmetries leads to a mutation in DNA, which could offer a new perspective in the scientific studies on the originand cause of mutation.