Utilization of Wood Based Hemicellulose Pre-Hydrolysate for the Production of High Value Added Platform Chemicals
Total Page:16
File Type:pdf, Size:1020Kb
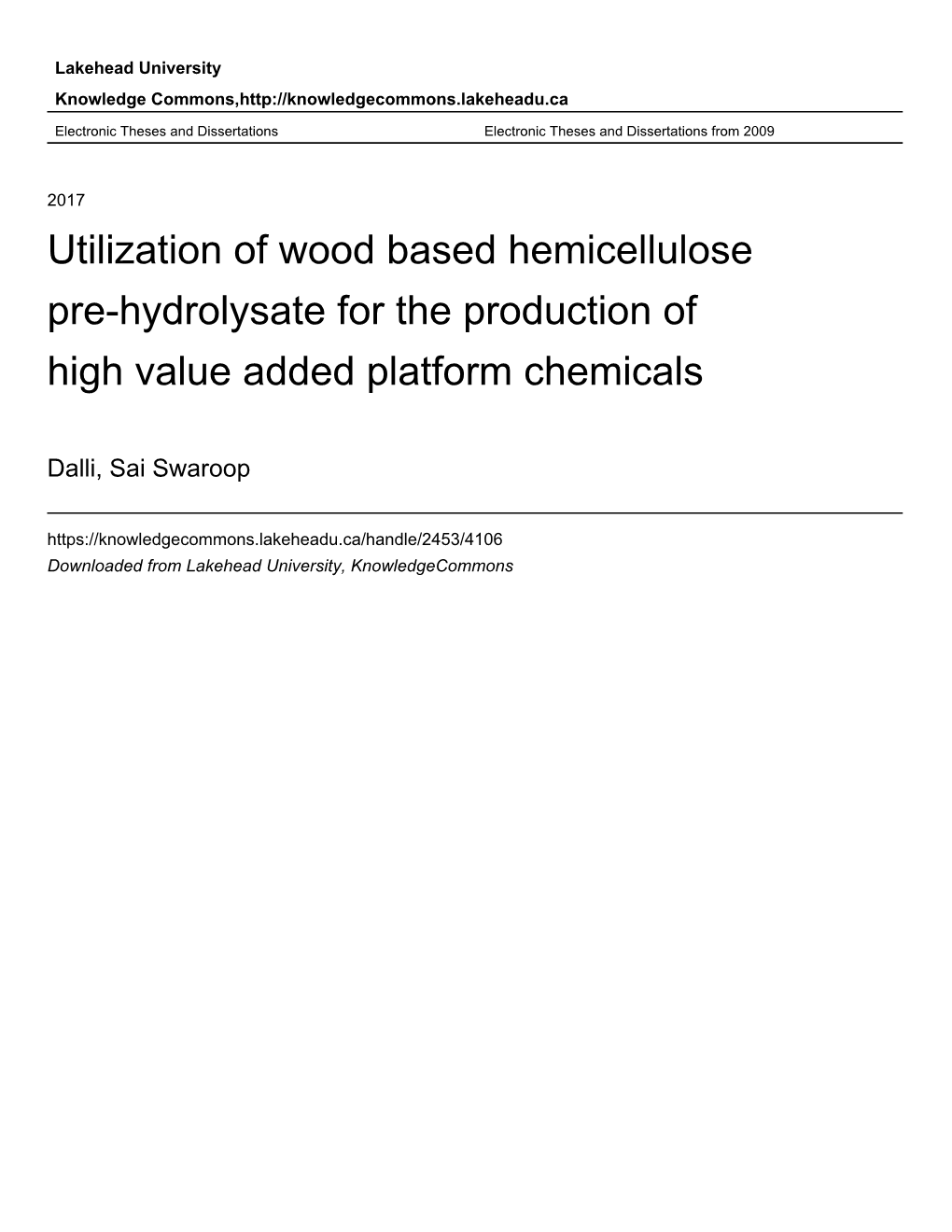
Load more
Recommended publications
-
PRODUCTION of ETHYL LEVULINATE VIA ESTERIFICATION REACTION of LEVULINIC ACID in the PRESENCE of Zro2 BASED CATALYST
Malaysian Journal of Analytical Sciences, Vol 23 No 1 (2019): 45 - 51 DOI: https://doi.org/10.17576/mjas-2019-2301-06 MALAYSIAN JOURNAL OF ANALYTICAL SCIENCES ISSN 1394 - 2506 Published by The Malaysian Analytical Sciences Society PRODUCTION OF ETHYL LEVULINATE VIA ESTERIFICATION REACTION OF LEVULINIC ACID IN THE PRESENCE OF ZrO2 BASED CATALYST (Penghasilan Etil Levulinat Melalui Pengesteran Asid Levulinik dengan Kehadiran Mangkin Berasaskan ZrO2) Dorairaaj Sivasubramaniam1, Nor Aishah Saidina Amin1*, Khairuddin Ahmad1, Nur Aainaa Syahirah Ramli2 1Chemical Reaction Engineering Group (CREG), Faculty of Chemical Engineering, Universiti Teknologi Malaysia, 81300 Skudai, Johor, Malaysia 2Advanced Oleochemical Technology Division, Malaysian Palm Oil Board (MPOB), 6, Persiaran Institusi, Bandar Baru Bangi, 43000 Kajang, Selangor, Malaysia *Corresponding author: [email protected] Received: 13 April 2017; Accepted: 17 April 2018 Abstract Ethyl levulinate is widely used as a fuel additive, flavor or fragrance and as a component of fuel blending. This study focused on the production of ethyl levulinate from levulinic acid via esterification reaction in the presence of HPW/ZrO2.The catalyst was prepared using the wet impregnation method, characterized by using FTIR, BET and NH3-TPD and screened based on 20%, 40% and 60% HPW/ZrO2. The 40% HPW/ZrO2 catalyst exhibited the highest catalytic performance during the parameter screening stage which included catalyst loading (0.25‒1.25g) and volume ratio of levulinic acid to ethanol (1:4 – 1:8). The highest ethyl levulinate yield of 99% corresponded to a catalyst loading of 0.5 g and volume ratio of levulinic acid to ethanol of 1:5 with reaction conditions at 150 °C for 3 hours. -
Production of Levulinic Acid from Cellulose and Cellulosic Biomass in Different Catalytic Systems
catalysts Review Production of Levulinic Acid from Cellulose and Cellulosic Biomass in Different Catalytic Systems Chen Liu 1, Xuebin Lu 1,2,*, Zhihao Yu 1 , Jian Xiong 2, Hui Bai 1 and Rui Zhang 3,* 1 School of Environmental Science and Engineering, Tianjin University, Tianjin 300350, China; [email protected] (C.L.); [email protected] (Z.Y.); [email protected] (H.B.) 2 Department of Chemistry & Environmental Science, School of Science, Tibet University, Lhasa 850000, China; [email protected] 3 School of Environmental and Municipal Engineering, Tianjin Chengjian University, Tianjin 300384, China * Correspondence: [email protected] (X.L.); [email protected] (R.Z.) Received: 2 August 2020; Accepted: 17 August 2020; Published: 3 September 2020 Abstract: The reasonable and effective use of lignocellulosic biomass is an important way to solve the current energy crisis. Cellulose is abundant in nature and can be hydrolyzed to a variety of important energy substances and platform compounds—for instance, glucose, 5-hydroxymethylfurfural (HMF), levulinic acid (LA), etc. As a chemical linker between biomass and petroleum processing, LA has become an ideal feedstock for the formation of liquid fuels. At present, some problems such as low yield, high equipment requirements, difficult separation, and serious environmental pollution in the production of LA from cellulose have still not been solved. Thus, a more efficient and green catalytic system of this process for industrial production is highly desired. Herein, we focus on the reaction mechanism, pretreatment, and catalytic systems of LA from cellulose and cellulosic biomass, and a series of existing technologies for producing LA are reviewed. -
Conversion of Furfuryl Alcohol Into Ethyl Levulinate Over Glucose-Derived Carbon-Based Solid Acid in Ethanol
molecules Article Conversion of Furfuryl Alcohol into Ethyl Levulinate over Glucose-Derived Carbon-Based Solid Acid in Ethanol Geng Zhao 1,*, Ming Liu 1, Xinkui Xia 2, Li Li 1 and Bayin Xu 1 1 Analysis and Testing Center, College of Chemistry and Chemical Engineering, Xinyang Normal University, Xinyang 464000, Henan, China; [email protected] (M.L.); [email protected] (L.L.); [email protected] (B.X.) 2 College of Food Science, Xinyang Agriculture and Forestry University, Xinyang 464000, Henan, China; [email protected] * Correspondence: [email protected]; Tel.: +86-376-639-3155 Received: 27 March 2019; Accepted: 15 May 2019; Published: 16 May 2019 Abstract: In this study, a carbon-based solid acid was created through the sulfonation of carbon obtained from the hydrothermal pretreatment of glucose. Additionally, ethyl levulinate, a viable liquid biofuel, was produced from furfuryl alcohol using the environmentally benign and low-cost catalyst in ethanol. Studies for optimizing the reaction conditions, such as reaction time, temperature, and catalyst loading, were performed. Under the optimal conditions, a maximum ethyl levulinate yield of 67.1% was obtained. The recovered catalyst activity (Ethyl levulinate yield 57.3%) remained high after being used four times, and it was easily regenerated with a simple sulfonation process. Moreover, the catalyst was characterized using FT-IR, XRD, SEM, elemental analysis, and acid-base titration techniques. Keywords: furfuryl alcohol; ethanol; ethyl levulinate; carbon-based solid acid 1. Introduction Because of the recent diminishment of fossil fuel resources, as well as environmental degradation resulting from greenhouse gas emissions, significant effort has been devoted to converting renewable biomass into liquid fuels, fuel additives, and organic bulk chemicals [1–3]. -
Direct Production of Ethyl Levulinate from Carbohydrates Catalyzed by H-ZSM-5 Supported Phosphotungstic Acid
PEER-REVIEWED ARTICLE bioresources.com Direct Production of Ethyl Levulinate from Carbohydrates Catalyzed by H-ZSM-5 Supported Phosphotungstic Acid Shiqiang Zhao,a Guizhuan Xu,b Junli Chang,a Chun Chang,a,c,* Jing Bai,a,c,* Shuqi Fang,a,c, and Ze Liu a A series of supported phosphotungstic acid (H3PW12O40, HPW) catalysts, including HPW/β, HPW/Sn-β, HPW/H-Y, HPW/H-ZSM-5, HPW/USY, HPW/ReUSY, and HPW/SBA-15, were prepared using an impregnation method for alcoholysis of fructose to ethyl levulinate in ethanol. Among these catalysts, HPW/H-ZSM-5 showed the highest catalytic activity, and the yield of ethyl levulinate from fructose increased with increasing phosphotungstic acid loading. The yield of ethyl levulinate reached 43.1% at 160 °C for 2 h over 20 wt.% HPW/H-ZSM-5, and the solid catalyst could be reused at least three times. EL yields of 19.1%, 27.3%, 37.4%, and 8.7% could be obtained from glucose, sucrose, inulin, and cellulose, respectively. Furthermore, the catalysts were characterized by BET surface area, X-ray diffraction, Fourier transform infrared spectroscopy, and X-ray photoelectron spectroscopy. HPW/H-ZSM-5 showed good catalytic activity for the direct production of ethyl levulinate from fructose. Keywords: Ethyl levulinate; Carbohydrates; H-ZSM-5; Phosphotungstic acid Contact information: a: School of Chemical Engineering and Energy, Zhengzhou University, Zhengzhou, 450001, China; b: College of Mechanical and Electrical Engineering, Henan Agricultural University, Zhengzhou, 450002, China; c: Engineering Laboratory of Henan Province for Biorefinery Technology and Equipment, Zhengzhou, 450001, China; * Corresponding authors: [email protected]; [email protected] INTRODUCTION Because of the depletion of fossil resources and increased environmental concerns, the development and utilization of renewable biomass resources has become an object of concern for countries around the world. -
Production of Bioadditive Ethyl Levulinate in the Catalytic Membrane
Ünlü & Hilmioğlu, JOTCSB. 2017;1(1):1–12. RESEARCH ARTICLE (This article was initially submitted to the UKMK 2016 (National Chemical Engineering Congress) and finally evaluated by the JOTCSB editorial staff). Production of Bioadditive Ethyl Levulinate in the Catalytic Membrane Reactor Derya Unlu1,* , Nilufer Hilmioglu 1 1 Kocaeli University, Engineering Faculty, Chemical Engineering Department, Umuttepe, Kocaeli, 41380, Turkey Abstract: Fuel bioadditive ethyl levulinate is the biofuel of the future. Reactants of ethyl levulinate are produced from biomass. Therefore, esterification of ethanol and levulinic acid is an environmentally friendly green process for the production of ethyl levulinate. In this study, synthesis of ethyl levulinate was carried out in the batch reactor and in the catalytic membrane reactor by using sulfated zirconia loaded catalytic membrane. Catalytic membrane reactor (CMR) has higher conversion values than the conventional batch reactor. Optimum operation conditions were specified as T=70°C, M=1:1, and C cat =8 g/L. The levulinic acid conversion reached 36 % and 89% in the batch reactor (BR) and in the CMR, respectively. Keywords: Bioadditive; Ethyl levulinate; Catalytic Membrane Reactor. Submitted: September 15, 2016 . Revised: October 05, 2016 . Accepted: November 02, 2016 . Cite this: Ünlü D, Hilmioğlu N. Production of Bioadditive Ethyl Levulinate in the Catalytic Membrane Reactor. JOTCSB. 2017;1(1):1–12. *Corresponding author. E-mail: [email protected]. 1 Ünlü & Hilmioğlu, JOTCSB. 2016;1(1):1–12. RESEARCH ARTICLE INTRODUCTION Global warming caused by greenhouse gases is known as one of the serious environmental problems in our age. As a result of the usage of fossil-derived fuels and also the reduction of the vegetation cover, the amount of carbon dioxide in the atmosphere has been increasing. -
Improved Catalytic Transfer Hydrogenation of Levulinate Esters with Alcohols Over Zro2 Catalyst †
Proceedings Improved Catalytic Transfer Hydrogenation of Levulinate Esters with Alcohols over ZrO2 Catalyst † Tommaso Tabanelli 1,*, Paola Blair Vásquez 1, Emilia Paone 2, Rosario Pietropaolo 2, Nikolaos Dimitratos 1, Fabrizio Cavani 1 and Francesco Mauriello 2 1 Dipartimento di Chimica Industriale “Toso Montanari”, Università di Bologna, Viale del Risorgimento 4, 40136 Bologna, Italy; [email protected] (P.B.V.); [email protected] (N.D.); [email protected] (F.C.) 2 Dipartimento DICEAM, Università Mediterranea di Reggio Calabria, Loc. Feo di Vito, 89122 Reggio Calabria, Italy; [email protected] (E.P.); [email protected] (R.P.); [email protected] (F.M.) * Correspondence: [email protected]; Tel.: +39-051-209-3861 † Presented at the 1st International Electronic Conference on Catalysis Sciences, 10–30 November 2020; Available online: https://eccs2020.sciforum.net. Published: 9 November 2020 Abstract: Levulinic acid (LA) and its esters (alkyl levulinates) are polyfunctional molecules that can be obtained from lignocellulosic biomass. Herein, the catalytic conversion of methyl and ethyl levulinates into γ-valerolactone (GVL) via catalytic transfer hydrogenation (CTH) by using methanol, ethanol, and 2-propanol as the H-donor/solvent, was investigated under both batch and gas-flow conditions. In particular, high-surface-area, tetragonal zirconia has proven to be a suitable catalyst for this reaction. Isopropanol was found to be the best H-donor under batch conditions, with ethyl levulinate providing the highest yield in GVL. However, long reaction times and high autogenic pressures are needed in order to work in the liquid-phase at high temperature with light alcohols. -
Novel Analysis on Aroma Compounds of Wine, Vinegar and Derived Products
Novel Analysis on Aroma Compounds of Wine, Vinegar and Derived Products Edited by Enrique Durán-Guerrero and Remedios Castro-Mejías Printed Edition of the Special Issue Published in Foods www.mdpi.com/journal/foods Novel Analysis on Aroma Compounds of Wine, Vinegar and Derived Products Novel Analysis on Aroma Compounds of Wine, Vinegar and Derived Products Editors Enrique Dur´an-Guerrero Remedios Castro-Mej´ıas MDPI Basel Beijing Wuhan Barcelona Belgrade Manchester Tokyo Cluj Tianjin • • • • • • • • • Editors Enrique Duran-Guerrero´ Remedios Castro-Mej´ıas University of Cadiz´ University of Cadiz´ Spain Spain Editorial Office MDPI St. Alban-Anlage 66 4052 Basel, Switzerland This is a reprint of articles from the Special Issue published online in the open access journal Foods (ISSN 2304-8158) (available at: https://www.mdpi.com/journal/foods/special issues/Wine Aroma). For citation purposes, cite each article independently as indicated on the article page online and as indicated below: LastName, A.A.; LastName, B.B.; LastName, C.C. Article Title. Journal Name Year, Volume Number, Page Range. ISBN 978-3-0365-0000-0 (Hbk) ISBN 978-3-0365-0000-0 (PDF) © 2021 by the authors. Articles in this book are Open Access and distributed under the Creative Commons Attribution (CC BY) license, which allows users to download, copy and build upon published articles, as long as the author and publisher are properly credited, which ensures maximum dissemination and a wider impact of our publications. The book as a whole is distributed by MDPI under the terms and conditions of the Creative Commons license CC BY-NC-ND. -
Catalytic Production of Levulinic Acid (LA) from Actual Biomass
molecules Review Catalytic Production of LevulinicLevulinic AcidAcid (LA)(LA) fromfrom ActualActual BiomassBiomass Michela SignorettoSignoretto, Somayeh, Somayeh Taghavi, Taghavi, Elena Elena Ghedini Ghedini and and Federica Federica Menegazzo Menegazzo * * CATMAT Lab,Lab, DepartmentDepartment ofof MolecularMolecular SciencesSciences andand Nanosystems,Nanosystems, Ca’ Foscari University of Venice and Ca’ Foscari University of Venice and INSTM RUVe, via Torino 155, 30172 Venezia Mestre, Italy INSTM RUVe, via Torino 155, 30172 Venezia Mestre, Italy * Correspondence: [email protected] * Correspondence: [email protected] Received: 28 June 2019; Accepted: 26 Ju Julyly 2019; Published: 30 30 July July 2019 2019 Abstract: Catalytic conversion of actual biomass to valuable chemicals is a crucial issue in green chemistry. This review discusses on the recent approachapproach in the levulinic acid (LA) formation from three prominent generationsgenerations ofof biomasses.biomasses. Our paper highlights the impact of the nature of different different types of biomass and their complex structure and im impurities,purities, different different groups of catalyst, solvents, and reaction system, and condition andand allall relatedrelated prospros andand conscons forfor thisthis process.process. Keywords: threethree generationsgenerations of of biomasses; biomasses; actual actual biomass biomass transformation; transformation; hydrolysis; hydrolysis; levulinic levulinic acid; catalysts;acid; catalysts; biorefinery biorefinery 1. Introduction Among thethe -
Ethyl Levulinate Synthesis from Levulinic Acid and Furfuryl Alcohol
547 A publication of CHEMICAL ENGINEERING TRANSACTIONS VOL. 78, 2020 The Italian Association of Chemical Engineering Online at www.cetjournal.it Guest Editors: Jeng Shiun Lim, Nor Alafiza Yunus, Jiří Jaromír Klemeš Copyright © 2020, AIDIC Servizi S.r.l. DOI: 10.3303/CET2078092 ISBN 978-88-95608-76-1; ISSN 2283-9216 Ethyl Levulinate Synthesis from Levulinic Acid and Furfuryl Alcohol by Using Modified Carbon Cryogels Muzakkir Mohammad Zainol, Wan Amri Nazreen, Persia Iskandar Ping Ylang, Tie Teck Hoe, Mohd Asmadi Mohammed Yussuf*, Nor Aishah Saidina Amin Chemical Reaction Engineering Group (CREG), School of Chemical and Energy Engineering, Faculty of Engineering, Universiti Teknologi Malaysia, 81310 Johor Bahru, Johor Darul Takzim, Malaysia [email protected] The research on ethyl levulinate synthesis is now increased due to its potential to be derived from biomass and for applications in biofuel. In the present work, model compound of biomass-derived intermediates, levulinic acid and furfural alcohol, were utilized for ethyl levulinate synthesis using modified carbon cryogel. Carbon cryogel produced from urea and furfural (UCC) mixtures was modified via sulfonation (UCC-S) and subsequently doped with Fe (UCC-S-Fe) to increase the surface chemistry of the reaction. In order to study the acidity and phase structure of the catalyst, the UCC-S and UCC-S-Fe were characterized by using NH3- TPD and XRD. The ethanolysis of levulinic acid and furfuryl alcohol were conducted in a batch reaction sy stem for the catalytic testing experiment by study the effect of reaction time and catalyst loading. At the selected conditions, with UCC-S as the catalyst, a high ethyl levulinate yield of 99.5 mol% was obtained from ethanolysis of levulinic acid using 10 wt% of a catalyst loading for 4 h. -
The Combustion Kinetics of the Lignocellulosic Biofuel, Ethyl Levulinate
Combustion and Flame 193 (2018) 157–169 Contents lists available at ScienceDirect Combustion and Flame journal homepage: www.elsevier.com/locate/combustflame The combustion kinetics of the lignocellulosic biofuel, ethyl levulinate ∗ Manik Kumer Ghosh a,b, , Mícheál Séamus Howard a,b, Yingjia Zhang c, Khalil Djebbi d, Gianluca Capriolo b, Aamir Farooq d, Henry J. Curran c, Stephen Dooley a,b a School of Physics, Trinity College Dublin, The University of Dublin, Ireland b Department of Chemical Sciences, University of Limerick, Ireland c Combustion Chemistry Centre, National University of Ireland, Galway, Ireland d Clean Combustion Research Center, King Abdullah University of Science and Technology, Thuwal, Saudi Arabia a r t i c l e i n f o a b s t r a c t Article history: Ethyl levulinate (Ethyl 4-oxopentanoate) is a liquid molecule at ambient temperature, comprising of ke- Received 2 November 2017 tone and ethyl ester functionalities and is one of the prominent liquid fuel candidates that may be eas- Revised 20 December 2017 ily obtained from lignocellulosic biomass. The combustion kinetics of ethyl levulinate have been investi- Accepted 28 February 2018 gated. Shock tube and rapid compression machine apparatuses are utilised to acquire gas phase ignition delay measurements of 0.5% ethyl levulinate/O 2 mixtures at φ = 1.0 and φ = 0.5 at ∼ 10 atm over the Keywords: temperature range 10 0 0–140 0 K. Ethyl levulinate is observed not to ignite at temperatures lower than Ethyl levulinate ∼1040 K in the rapid compression machine. The shock tube and rapid compression machine data are Lignocellulosic biofuel closely consistent and show ethyl levulinate ignition delay to exhibit an Arrhenius dependence to temper- Kinetic model ature. -
Downloaded 9/30/2021 2:22:38 AM
Reaction Chemistry & Engineering View Article Online PAPER View Journal | View Issue Bifunctional carbon Ni/NiO nanofiber catalyst Cite this: React. Chem. Eng.,2020, based on 5-sulfosalicylic acid for conversion of 5,1759 C5/C6 carbohydrates into ethyl levulinate† Haixin Guo, *a Yuya Abe, a Xinhua Qi b and Richard Lee Smith Jr *ac A method was developed for preparing bifunctional carbon Ni/NiO nanofiber catalysts that promote efficient one-pot conversion of C5/C6 carbohydrates into levulinate esters in alcohol solvents. The bifunctional catalysts were prepared via solvothermal carbonization of 5-sulfosalicylic acid/NiSO4 without the use of sulfuric acid or hydrogen gas and had fine particle sizes (d = 5 nm to 50 nm) and contained –NH2, –SO3H, –COOH and phenolic –OH functional groups. Under optimal conditions, the catalysts afforded 93% selectivity of ethyl levulinate in ethanol with the major intermediate being 2-(ethoxymethyl) furan, 4,5,5-triethoxypentan-2-one and major byproduct being 2,5,5-triethoxpentan-2-one. Cooperative Received 19th April 2020, activity of Lewis acidity, Brønsted acidity and functional group sites of the catalyst is demonstrated for Accepted 30th July 2020 multi-step reaction sequences of C5/C6 carbohydrates with one-pot conversions and alcohols (methanol, ethanol, 1-propanol, 1-butanol) that act as both solvent and hydrogen donor source in which the DOI: 10.1039/d0re00153h bifunctional catalyst was shown to be recyclable five times with no apparent change in conversion and ca. rsc.li/reaction-engineering 5% change in selectivity. 1. Introduction advantageously to avoid intermediate separation and purification steps for targeted products. -
A Review on the Conversion of Levulinic Acid and Its Esters to Various Useful Chemicals
AIMS Energy, 7(2): 165–185. DOI: 10.3934/energy.2019.2.165 Received: 28 January 2019 Accepted: 28 March 2019 Published: 17 April 2019 http://www.aimspress.com/journal/energy Review A Review on the conversion of levulinic acid and its esters to various useful chemicals Aderemi T. Adeleye1, Hitler Louis2,4,*, Ozioma U. Akakuru3,4, Innocent Joseph2, Obieze C. Enudi4 and Dass P. Michael5 1 Dalian Institute of Chemical Physics, Chinese Academy of Sciences, Dalian 116023, P. R. China 2 CAS Key Laboratory for Nanosystem and Hierarchical Fabrication, CAS Centre for Excellence in Nanoscience, National Centre for Nanoscience and Technology, University of Chinese Academy of Sciences, Beijing, China 3 Ningbo Institute of Materials Technology and Engineering, Chinese Academy of Sciences, Zhejiang, China 4 Department of Pure and Applied Chemistry, Faculty of Physical Sciences, University of Calabar, Calabar, Nigeria 5 Department of Chemistry, Modibbo Adama University of Technology, Yola, Nigeria * Correspondence: Email: [email protected]; [email protected]. Abstract: Levulinic acid (LA), an important chemical produced from a bio-based resource for current petrochemical operation, the details of hydrogenation to gamma (γ)-valerolactone (GVL) is reviewed. Levulinic acid (LA) was listed among one of the top value-added chemicals by U.S. Department of Energy and also been identified as a promising sustainable material for the synthesis of other important chemicals. It can be synthesized via a process known as hydrolysis. Its synthetic hydrolysis can be carried out employing some kinds of saccharides (e.g. glucose), the major constituent unit composed in cellulose. Its production from cellulose, the most abundant and renewable natural resource on earth is advantageous; however, recalcitrance nature that holds components together in biomass prevents the easy accessibility to the utilization of cellulose therefore as a result of this, considerable pretreatment is required.