Analysis of the Role of Two Autophagy Pathway Related Genes, Becn1 and Tsc1, in Murine Mammary Gland Development and Differentiation
Total Page:16
File Type:pdf, Size:1020Kb
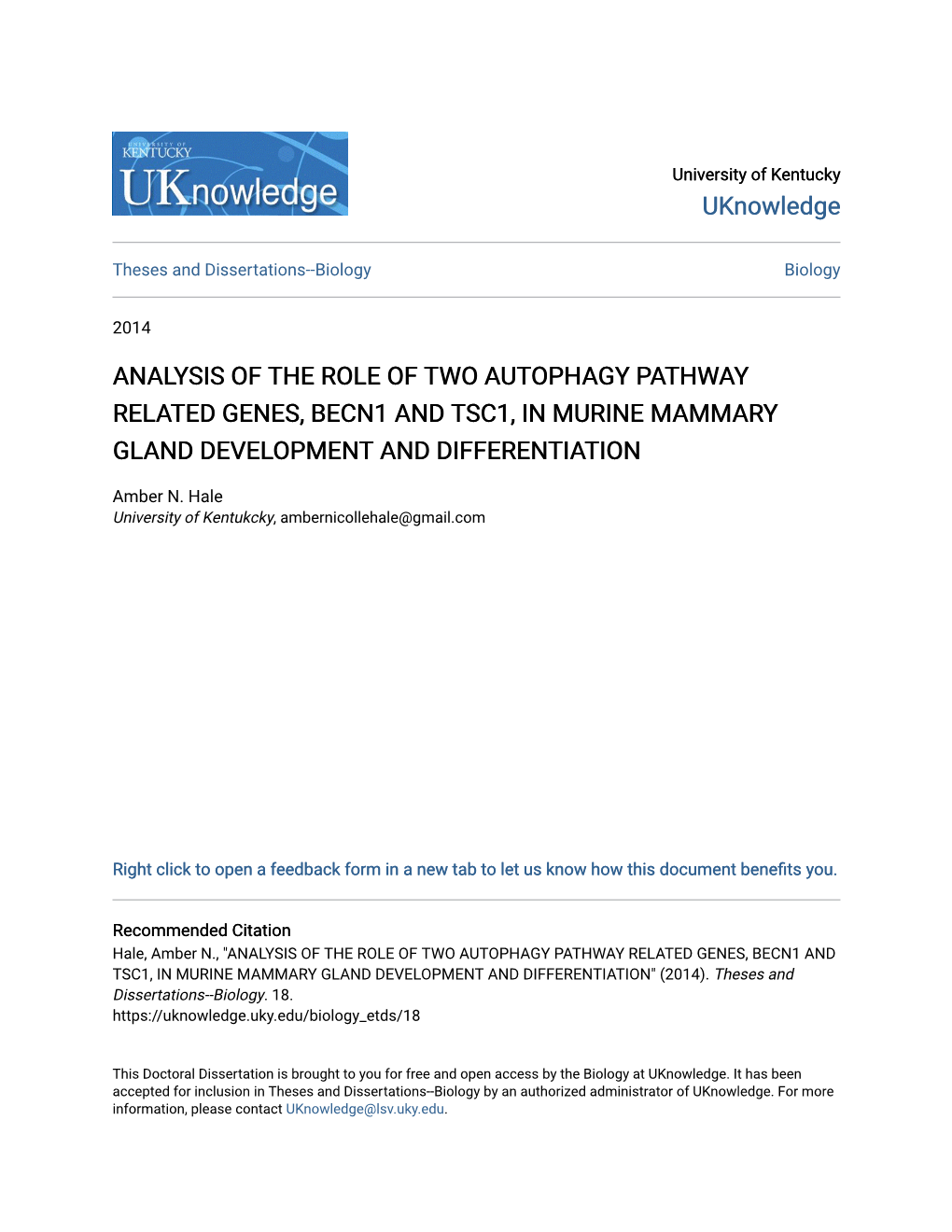
Load more
Recommended publications
-
Autophagy Patterns and Prognosis in Uveal Melanomas
Modern Pathology (2011) 24, 1036–1045 1036 & 2011 USCAP, Inc. All rights reserved 0893-3952/11 $32.00 Autophagy patterns and prognosis in uveal melanomas Alexandra N Giatromanolaki1, Georgios St Charitoudis2, Nikolaos E Bechrakis3, Vassilios P Kozobolis4, Michael I Koukourakis5, Michael H Foerster2 and Efthimios L Sivridis1 1Department of Pathology, Democritus University of Thrace Medical School, Alexandroupolis, Greece; 2Department of Ophthalmology, Charite´ Medical University, Campus Benjamin Franklin, Berlin, Germany; 3Department of Ophthalmology, University of Innsbruck Medical School, Innsbruck, Austria; 4Department of Ophthalmology, Democritus University of Thrace Medical School, Alexandroupolis, Greece and 5Department of Radiotherapy/Oncology, Democritus University of Thrace Medical School, Alexandroupolis, Greece Autophagy is a self-degradation mechanism by which cells recycle their own cytoplasmic constituents. It has been claimed that, under certain conditions, such a process may be associated with tumor progression. In this study, the autophagic activity was investigated in a series of 99 uveal melanomas after immunohistochemical staining for the autophagy-associated proteins MAP1LC3A and BECN1, most commonly known as LC3A and Beclin 1, respectively. These were assessed in parallel with the hypoxia-inducible factor 1a (HIF1A) and its downstream protein lactate dehydrogenase 5 (composed by five LDHA subunits). Increased autophagic reactivity, detected by MAP1LC3A or BECN1, was associated with intense pigmentation and tumor hypoxia. Uveal melanomas with extensive overexpression of BECN1 or those with underexpression of this protein were associated with the worst prognosis, but the former manifested metastases much earlier than the latter; only 58% of patients with extensive BECN1 overexpression were alive at 4 years, compared with 80% of patients with underexpressed patterns. -
Target-Derived Neurotrophins Coordinate Transcription and Transport of Bclw to Prevent Axonal Degeneration
The Journal of Neuroscience, March 20, 2013 • 33(12):5195–5207 • 5195 Neurobiology of Disease Target-Derived Neurotrophins Coordinate Transcription and Transport of Bclw to Prevent Axonal Degeneration Katharina E. Cosker,1,2,3 Maria F. Pazyra-Murphy,1,2,3 Sara J. Fenstermacher,1,2,3 and Rosalind A. Segal1,2,3 1Department of Neurobiology, Harvard Medical School, Boston, Massachusetts 02115, and Departments of 2Cancer Biology and 3Pediatric Oncology, Dana- Farber Cancer Institute, Boston, Massachusetts 02215 Establishment of neuronal circuitry depends on both formation and refinement of neural connections. During this process, target- derived neurotrophins regulate both transcription and translation to enable selective axon survival or elimination. However, it is not known whether retrograde signaling pathways that control transcription are coordinated with neurotrophin-regulated actions that transpire in the axon. Here we report that target-derived neurotrophins coordinate transcription of the antiapoptotic gene bclw with transport of bclw mRNA to the axon, and thereby prevent axonal degeneration in rat and mouse sensory neurons. We show that neurotrophin stimulation of nerve terminals elicits new bclw transcripts that are immediately transported to the axons and translated into protein. Bclw interacts with Bax and suppresses the caspase6 apoptotic cascade that fosters axonal degeneration. The scope of bclw regulation at the levels of transcription, transport, and translation provides a mechanism whereby sustained neurotrophin stimulation -
Transient Unfolding and Long-Range Interactions in Viral BCL2 M11 Enable Binding to the BECN1 BH3 Domain
biomolecules Article Transient Unfolding and Long-Range Interactions in Viral BCL2 M11 Enable Binding to the BECN1 BH3 Domain Arvind Ramanathan 1,2,*, Akash Parvatikar 3, Srinivas C. Chennubhotla 3,* and Yang Mei 4,† and Sangita C. Sinha 4,* 1 Data Science and Learning Division, Argonne National Laboratory, Lemont, IL 60439, USA 2 Consortium for Advanced Science and Engineering, University of Chicago, Chicago, IL 60637, USA 3 Department of Computational and Systems Biology, University of Pittsburgh, PA 15260, USA; [email protected] 4 Department of Chemistry and Biochemistry, North Dakota State University, Fargo, ND 58108, USA; [email protected] * Correspondence: [email protected] (A.R.); [email protected] (S.C.C.); [email protected] (S.C.S.); Tel.: +1-630-252-3805 (A.R.); +1-412-648-7794 (S.C.C.); +1-701-231-5658 (S.C.S.) † Current address: The Wistar Institute, Philadelphia, PA 19104, USA. Received: 27 July 2020; Accepted: 4 September 2020; Published: 11 September 2020 Abstract: Viral BCL2 proteins (vBCL2s) help to sustain chronic infection of host proteins to inhibit apoptosis and autophagy. However, details of conformational changes in vBCL2s that enable binding to BH3Ds remain unknown. Using all-atom, multiple microsecond-long molecular dynamic simulations (totaling 17 µs) of the murine g-herpesvirus 68 vBCL2 (M11), and statistical inference techniques, we show that regions of M11 transiently unfold and refold upon binding of the BH3D. Further, we show that this partial unfolding/refolding within M11 is mediated by a network of hydrophobic interactions, which includes residues that are 10 Å away from the BH3D binding cleft. -
Celebrating Student Research, Scholarship, and Creativity April 23, 2019 Annual Kirkland/Spizuoco Memorial Science Lecture Dr
SHIPPENSBURG UNIVERSITY CELEBRATING STUDENT RESEARCH, SCHOLARSHIP, AND CREATIVITY APRIL 23, 2019 ANNUAL KIRKLAND/SPIZUOCO MEMORIAL SCIENCE LECTURE DR. CHAD ORZEL MONDAY, APRIL 22 3:30PM ● LUHRS PERFORMING ARTS CENTER DISCOVERING YOUR INNER SCIENTIST This lecture, honors Dr. Gordon L. Kirkland Jr., professor of biology, and Dr. Joseph A. Spizuoco, professor of physics for their dedication and commitment to Shippensburg University students and their respective academic fields. In the popular imagination, science is a collection of arcane facts that only a minority of people are capable of understanding. In reality, science is a process for generating knowledge by looking at the world, thinking of possible explanations for interesting phenomena, testing those models by observation and experiment, and telling the results of those tests to others. This process is an essential human activity. This talk explains how everyday activities like card games, crossword puzzles, and sports make use of the same mental tools scientists have used to revolutionize our understanding of the universe. Chad Orzel is a professor at Union College in Schenectady, New York, and the author of four books explaining science for non-scientists: How to Teach Quantum Physics to Your Dog and How to Teach Relativity to Your Dog, which explain modern physics through imaginary conversations with Emmy, his German shepherd, and Eureka: Discovering Your Inner Scientist, on the role of scientific thinking in everyday life. His latest book, Breakfast with Einstein: The Exotic Physics of Everyday Objects, explains how quantum phenomena manifest during the course of ordinary morning activities. He has a BA in physics from Williams College and a PhD in chemical physics from the University of Maryland, College Park, where he did his thesis research on collisions of laser-cooled atoms at the National Institute of Standards and Technology in the lab of Bill Phillips. -
Mitophagy Confers Resistance to Siderophore-Mediated Killing by Pseudomonas Aeruginosa
Mitophagy confers resistance to siderophore-mediated killing by Pseudomonas aeruginosa Natalia V. Kirienkoa,b, Frederick M. Ausubela,b,1, and Gary Ruvkuna,b,1,2 aDepartment of Molecular Biology, Massachusetts General Hospital, Boston, MA 02114; and bDepartment of Genetics, Harvard Medical School, Boston, MA 02115 Contributed by Gary Ruvkun, December 31, 2014 (sent for review December 19, 2014) In the arms race of bacterial pathogenesis, bacteria produce an Results and Discussion array of toxins and virulence factors that disrupt core host Pyoverdin Enters C. elegans and Is Sufficient to Mediate Host Killing. processes. Hosts mitigate the ensuing damage by responding with Despite the presence of rich sources of iron within host cells, immune countermeasures. The iron-binding siderophore pyover- siderophores are generally assumed to scavenge iron from fer- din is a key virulence mediator of the human pathogen Pseudo- riproteins present in the extracellular milieu. However, we hy- monas aeruginosa, but its pathogenic mechanism has not been pothesize that siderophores are capable of harvesting iron from established. Here we demonstrate that pyoverdin enters Caen- intracellular sources and, consequently, function directly as orhabditis elegans and that it is sufficient to mediate host killing. toxins. We exposed worms to a pyoverdin-enriched, cell-free Moreover, we show that iron chelation disrupts mitochondrial ho- bacterial growth media for 24 h to determine whether detectable meostasis and triggers mitophagy both in C. elegans and mamma- levels of pyoverdin could be identified within the host. After lian cells. Finally, we show that mitophagy provides protection exposure, worms were washed extensively, homogenized, and both against the extracellular pathogen P. -
Genome-Wide Meta-Analysis of Common Variant Differences Between Men and Women
Human Molecular Genetics, 2012, Vol. 21, No. 21 4805–4815 doi:10.1093/hmg/dds304 Advance Access published on July 27, 2012 Genome-wide meta-analysis of common variant differences between men and women Vesna Boraska1,2,∗, Ana Jeroncˇic´ 3, Vincenza Colonna1,5, Lorraine Southam1, Dale R. Nyholt 6, Nigel William Rayner1,7,8, John R.B. Perry7,9,10, Daniela Toniolo11, Eva Albrecht12, Wei Ang13, StefaniaBandinelli14, MajaBarbalic15, IneˆsBarroso1,16, Jacques S.Beckmann18,19, ReinerBiffar20, Dorret Boomsma22, Harry Campbell23, Tanguy Corre11, Jeanette Erdmann24,25,To˜ nu Esko27,28,29, Krista Fischer27, Nora Franceschini30, Timothy M. Frayling9, Giorgia Girotto31, Juan R. Gonzalez32,33, Tamara B. Harris34, Andrew C. Heath35, Iris M. Heid36,37, Wolfgang Hoffmann21, Albert Hofman38,40, Momoko Horikoshi7,8, Jing Hua Zhao17, Anne U. Jackson41,42, Jouke-Jan Hottenga22, Antti Jula43, Mika Ka¨ho¨ nen44,46, Kay-Tee Khaw47, Lambertus A. Kiemeney48, Norman Klopp52, Zolta´n Kutalik18,54, Vasiliki Lagou7,8, Downloaded from Lenore J.Launer34, TerhoLehtima¨ki45,46, Mathieu Lemire55, Marja-LiisaLokki56, Christina Loley26, Jian’an Luan17, Massimo Mangino10, Irene Mateo Leach58, Sarah E. Medland6, Evelin Mihailov28, Grant W. Montgomery6, Gerjan Navis59, John Newnham13, Markku S. Nieminen61, Aarno Palotie1,57,62,63, Kalliope Panoutsopoulou1, Annette Peters53, Nicola Pirastu31, http://hmg.oxfordjournals.org/ Ozren Polasˇek4, Karola Rehnstro¨ m1,57, Samuli Ripatti57, Graham R.S. Ritchie1,64, Fernando Rivadeneira38,39,40, Antonietta Robino31, Nilesh J. Samani65, So-Youn Shin1, Juha Sinisalo61, Johannes H. Smit66, Nicole Soranzo1,10, Lisette Stolk39,40, Dorine W. Swinkels49, Toshiko Tanaka68, Alexander Teumer69, Anke To¨ njes70,71, Michela Traglia11, Jaakko Tuomilehto72,73,74,75, Armand Valsesia18,54,76, Wiek H. -
Supplementary Table 2
Supplementary Table 2. Differentially Expressed Genes following Sham treatment relative to Untreated Controls Fold Change Accession Name Symbol 3 h 12 h NM_013121 CD28 antigen Cd28 12.82 BG665360 FMS-like tyrosine kinase 1 Flt1 9.63 NM_012701 Adrenergic receptor, beta 1 Adrb1 8.24 0.46 U20796 Nuclear receptor subfamily 1, group D, member 2 Nr1d2 7.22 NM_017116 Calpain 2 Capn2 6.41 BE097282 Guanine nucleotide binding protein, alpha 12 Gna12 6.21 NM_053328 Basic helix-loop-helix domain containing, class B2 Bhlhb2 5.79 NM_053831 Guanylate cyclase 2f Gucy2f 5.71 AW251703 Tumor necrosis factor receptor superfamily, member 12a Tnfrsf12a 5.57 NM_021691 Twist homolog 2 (Drosophila) Twist2 5.42 NM_133550 Fc receptor, IgE, low affinity II, alpha polypeptide Fcer2a 4.93 NM_031120 Signal sequence receptor, gamma Ssr3 4.84 NM_053544 Secreted frizzled-related protein 4 Sfrp4 4.73 NM_053910 Pleckstrin homology, Sec7 and coiled/coil domains 1 Pscd1 4.69 BE113233 Suppressor of cytokine signaling 2 Socs2 4.68 NM_053949 Potassium voltage-gated channel, subfamily H (eag- Kcnh2 4.60 related), member 2 NM_017305 Glutamate cysteine ligase, modifier subunit Gclm 4.59 NM_017309 Protein phospatase 3, regulatory subunit B, alpha Ppp3r1 4.54 isoform,type 1 NM_012765 5-hydroxytryptamine (serotonin) receptor 2C Htr2c 4.46 NM_017218 V-erb-b2 erythroblastic leukemia viral oncogene homolog Erbb3 4.42 3 (avian) AW918369 Zinc finger protein 191 Zfp191 4.38 NM_031034 Guanine nucleotide binding protein, alpha 12 Gna12 4.38 NM_017020 Interleukin 6 receptor Il6r 4.37 AJ002942 -
Phenotype-Based Drug Screening Reveals Association Between Venetoclax Response and Differentiation Stage in Acute Myeloid Leukemia
Acute Myeloid Leukemia SUPPLEMENTARY APPENDIX Phenotype-based drug screening reveals association between venetoclax response and differentiation stage in acute myeloid leukemia Heikki Kuusanmäki, 1,2 Aino-Maija Leppä, 1 Petri Pölönen, 3 Mika Kontro, 2 Olli Dufva, 2 Debashish Deb, 1 Bhagwan Yadav, 2 Oscar Brück, 2 Ashwini Kumar, 1 Hele Everaus, 4 Bjørn T. Gjertsen, 5 Merja Heinäniemi, 3 Kimmo Porkka, 2 Satu Mustjoki 2,6 and Caroline A. Heckman 1 1Institute for Molecular Medicine Finland, Helsinki Institute of Life Science, University of Helsinki, Helsinki; 2Hematology Research Unit, Helsinki University Hospital Comprehensive Cancer Center, Helsinki; 3Institute of Biomedicine, School of Medicine, University of Eastern Finland, Kuopio, Finland; 4Department of Hematology and Oncology, University of Tartu, Tartu, Estonia; 5Centre for Cancer Biomarkers, De - partment of Clinical Science, University of Bergen, Bergen, Norway and 6Translational Immunology Research Program and Department of Clinical Chemistry and Hematology, University of Helsinki, Helsinki, Finland ©2020 Ferrata Storti Foundation. This is an open-access paper. doi:10.3324/haematol. 2018.214882 Received: December 17, 2018. Accepted: July 8, 2019. Pre-published: July 11, 2019. Correspondence: CAROLINE A. HECKMAN - [email protected] HEIKKI KUUSANMÄKI - [email protected] Supplemental Material Phenotype-based drug screening reveals an association between venetoclax response and differentiation stage in acute myeloid leukemia Authors: Heikki Kuusanmäki1, 2, Aino-Maija -
BCL2L2 (NM 004050) Human Tagged ORF Clone – RC211152 | Origene
OriGene Technologies, Inc. 9620 Medical Center Drive, Ste 200 Rockville, MD 20850, US Phone: +1-888-267-4436 [email protected] EU: [email protected] CN: [email protected] Product datasheet for RC211152 BCL2L2 (NM_004050) Human Tagged ORF Clone Product data: Product Type: Expression Plasmids Product Name: BCL2L2 (NM_004050) Human Tagged ORF Clone Tag: Myc-DDK Symbol: BCL2L2 Synonyms: BCL-W; BCL2-L-2; BCLW; PPP1R51 Vector: pCMV6-Entry (PS100001) E. coli Selection: Kanamycin (25 ug/mL) Cell Selection: Neomycin ORF Nucleotide >RC211152 ORF sequence Sequence: Red=Cloning site Blue=ORF Green=Tags(s) TTTTGTAATACGACTCACTATAGGGCGGCCGGGAATTCGTCGACTGGATCCGGTACCGAGGAGATCTGCC GCCGCGATCGCC ATGGCGACCCCAGCCTCGGCCCCAGACACACGGGCTCTGGTGGCAGACTTTGTAGGTTATAAGCTGAGGC AGAAGGGTTATGTCTGTGGAGCTGGCCCCGGGGAGGGCCCAGCAGCTGACCCGCTGCACCAAGCCATGCG GGCAGCTGGAGATGAGTTCGAGACCCGCTTCCGGCGCACCTTCTCTGATCTGGCGGCTCAGCTGCATGTG ACCCCAGGCTCAGCCCAACAACGCTTCACCCAGGTCTCCGATGAACTTTTTCAAGGGGGCCCCAACTGGG GCCGCCTTGTAGCCTTCTTTGTCTTTGGGGCTGCACTGTGTGCTGAGAGTGTCAACAAGGAGATGGAACC ACTGGTGGGACAAGTGCAGGAGTGGATGGTGGCCTACCTGGAGACGCGGCTGGCTGACTGGATCCACAGC AGTGGGGGCTGGGCGGAGTTCACAGCTCTATACGGGGACGGGGCCCTGGAGGAGGCGCGGCGTCTGCGGG AGGGGAACTGGGCATCAGTGAGGACAGTGCTGACGGGGGCCGTGGCACTGGGGGCCCTGGTAACTGTAGG GGCCTTTTTTGCTAGCAAG ACGCGTACGCGGCCGCTCGAGCAGAAACTCATCTCAGAAGAGGATCTGGCAGCAAATGATATCCTGGATT ACAAGGATGACGACGATAAGGTTTAA Protein Sequence: >RC211152 protein sequence Red=Cloning site Green=Tags(s) MATPASAPDTRALVADFVGYKLRQKGYVCGAGPGEGPAADPLHQAMRAAGDEFETRFRRTFSDLAAQLHV TPGSAQQRFTQVSDELFQGGPNWGRLVAFFVFGAALCAESVNKEMEPLVGQVQEWMVAYLETRLADWIHS -
Alterations of the Interactome of Bcl-2 Proteins in Breast Cancer at the Transcriptional, Mutational and Structural Level
RESEARCH ARTICLE Alterations of the interactome of Bcl-2 proteins in breast cancer at the transcriptional, mutational and structural level Simon Mathis Kønig1, Vendela Rissler1, Thilde Terkelsen1, Matteo Lambrughi1, 1,2 Elena PapaleoID * 1 Computational Biology Laboratory, Danish Cancer Society Research Center, Copenhagen, Denmark, a1111111111 2 Translational Disease Systems Biology, Faculty of Health and Medical Sciences, Novo Nordisk Foundation Center for Protein Research University of Copenhagen, Copenhagen, Denmark a1111111111 a1111111111 * [email protected] a1111111111 a1111111111 Abstract Apoptosis is an essential defensive mechanism against tumorigenesis. Proteins of the B- OPEN ACCESS cell lymphoma-2 (Bcl-2) family regulate programmed cell death by the mitochondrial apopto- sis pathway. In response to intracellular stress, the apoptotic balance is governed by inter- Citation: Kønig SM, Rissler V, Terkelsen T, Lambrughi M, Papaleo E (2019) Alterations of the actions of three distinct subgroups of proteins; the activator/sensitizer BH3 (Bcl-2 homology interactome of Bcl-2 proteins in breast cancer at 3)-only proteins, the pro-survival, and the pro-apoptotic executioner proteins. Changes in the transcriptional, mutational and structural level. expression levels, stability, and functional impairment of pro-survival proteins can lead to an PLoS Comput Biol 15(12): e1007485. https://doi. imbalance in tissue homeostasis. Their overexpression or hyperactivation can result in org/10.1371/journal.pcbi.1007485 oncogenic effects. Pro-survival Bcl-2 family members carry out their function by binding the Editor: Igor N. Berezovsky, A�STAR Singapore, BH3 short linear motif of pro-apoptotic proteins in a modular way, creating a complex net- SINGAPORE work of protein-protein interactions. Their dysfunction enables cancer cells to evade cell Received: July 8, 2019 death. -
Biology of the Caenorhabditis Elegans Germline Stem Cell System
| WORMBOOK CELL FATE, SIGNALING, AND DEVELOPMENT Biology of the Caenorhabditis elegans Germline Stem Cell System E. Jane Albert Hubbard*,1 and Tim Schedl†,1 *Skirball Institute of Biomolecular Medicine, Departments of Cell Biology and Pathology, New York University School of Medicine, † New York 10016 and Department of Genetics, Washington University School of Medicine, St. Louis, Missouri 63110 ORCID IDs: 0000-0001-5893-7232 (E.J.A.H.); 0000-0003-2148-2996 (T.S.) ABSTRACT Stem cell systems regulate tissue development and maintenance. The germline stem cell system is essential for animal reproduction, controlling both the timing and number of progeny through its influence on gamete production. In this review, we first draw general comparisons to stem cell systems in other organisms, and then present our current understanding of the germline stem cell system in Caenorhabditis elegans. In contrast to stereotypic somatic development and cell number stasis of adult somatic cells in C. elegans, the germline stem cell system has a variable division pattern, and the system differs between larval development, early adult peak reproduction and age-related decline. We discuss the cell and developmental biology of the stem cell system and the Notch regulated genetic network that controls the key decision between the stem cell fate and meiotic development, as it occurs under optimal laboratory conditions in adult and larval stages. We then discuss alterations of the stem cell system in response to environ- mental perturbations and aging. A recurring distinction is between processes that control stem cell fate and those that control cell cycle regulation. C. elegans is a powerful model for understanding germline stem cells and stem cell biology. -
Beclin-1 Expression Is Retained in High-Grade Serous Ovarian Cancer Yet Is Not Essential for Autophagy Induction in Vitro Rohann J
Western University Scholarship@Western Biochemistry Publications Biochemistry Department 8-2015 Beclin-1 Expression is Retained in High-Grade Serous Ovarian Cancer yet is Not Essential for Autophagy Induction In Vitro Rohann J. M. Correa Western University Yudith Ramos Valdes London Regional Cancer Program Trevor G. Shepherd Western University Gabriel E. DiMattia Western University, [email protected] Follow this and additional works at: https://ir.lib.uwo.ca/biochempub Part of the Biochemistry Commons Citation of this paper: Correa, Rohann J. M.; Valdes, Yudith Ramos; Shepherd, Trevor G.; and DiMattia, Gabriel E., "Beclin-1 Expression is Retained in High- Grade Serous Ovarian Cancer yet is Not Essential for Autophagy Induction In Vitro" (2015). Biochemistry Publications. 166. https://ir.lib.uwo.ca/biochempub/166 Correa et al. Journal of Ovarian Research (2015) 8:52 DOI 10.1186/s13048-015-0182-y RESEARCH Open Access Beclin-1 expression is retained in high-grade serous ovarian cancer yet is not essential for autophagy induction in vitro Rohann J. M. Correa1,2, Yudith Ramos Valdes1, Trevor G. Shepherd1,3,4,5† and Gabriel E. DiMattia1,2,3,4,6*† Abstract Background: Autophagy is a conserved cellular self-digestion mechanism that can either suppress or promote cancer in a context-dependent manner. In ovarian cancer, prevalent mono-allelic deletion of BECN1 (a canonical autophagy-inducer) suggests that autophagy is impaired to promote carcinogenesis and that Beclin-1 is a haploinsufficient tumor suppressor. Nonetheless, autophagy is known to be readily inducible in ovarian cancer cells. We sought to clarify whether Beclin-1 expression is in fact disrupted in ovarian cancer and whether this impacts autophagy regulation.