PDF (Chapter 5)
Total Page:16
File Type:pdf, Size:1020Kb
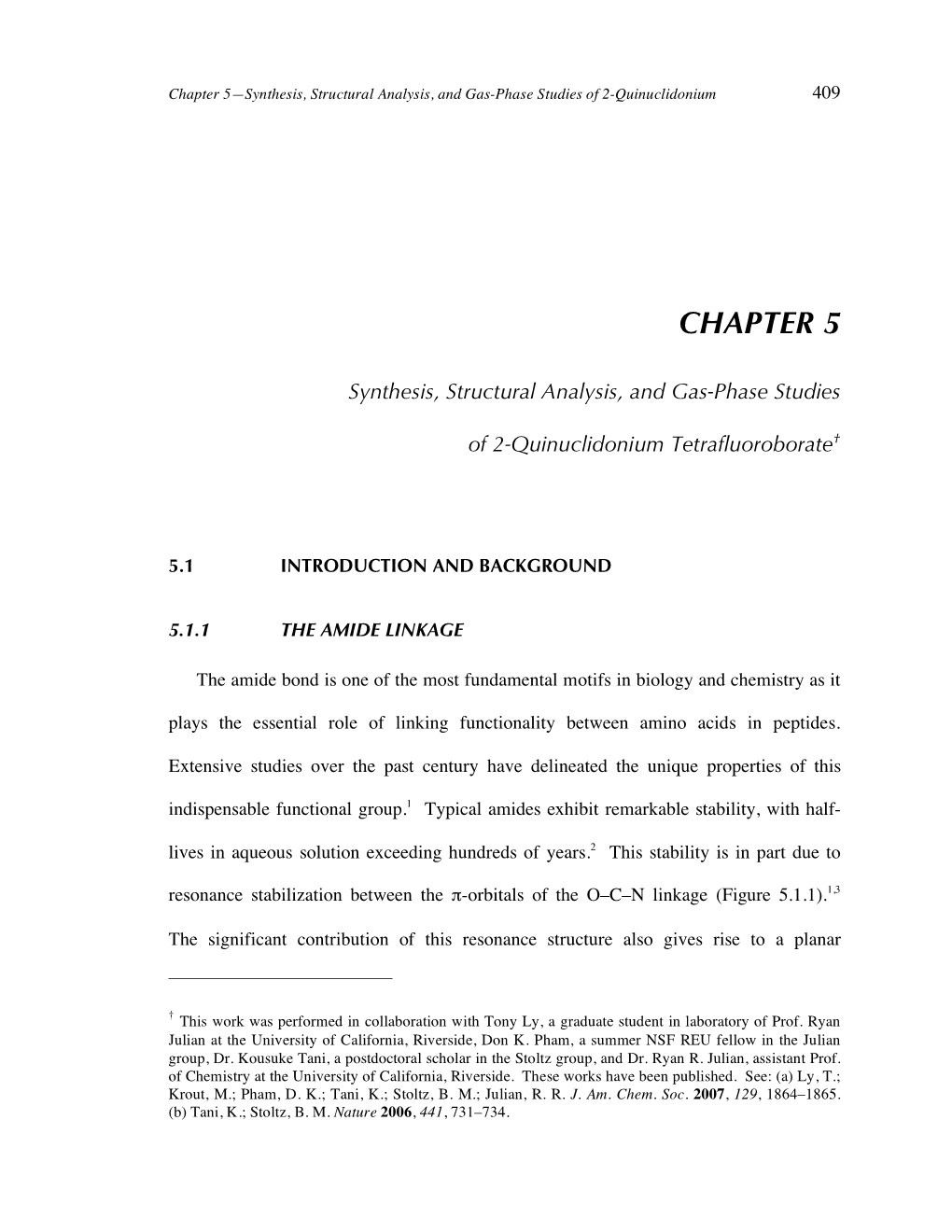
Load more
Recommended publications
-
779 Part 770—Interpretations
Pt. 770 15 CFR Ch. VII (1–1–21 Edition) in the item that qualitatively affect the per- roller bearings and parts). This applies formance of the U.S. and foreign items; to separate shipments of anti-friction (vi) Evidence of the interchangeability of bearings or bearing systems and anti- U.S. and foreign items; friction bearings or bearing systems (vii) Patent descriptions for the U.S. and foreign items; shipped with machinery or equipment (viii) Evidence that the U.S. and foreign for which they are intended to be used items meet a published industry, national, or as spares or replacement parts. international standard; (2) An anti-friction bearing or bear- (ix) A report or eyewitness account, by ing system physically incorporated in a deposition or otherwise, of the foreign item’s segment of a machine or in a complete operation; machine prior to shipment loses its (x) Evidence concerning the foreign manu- identity as a bearing. In this scenario, facturers’ corporate reputation; (xi) Comparison of the U.S. and foreign end the machine or segment of machinery item(s) made from a specific commodity, containing the bearing is the item sub- tool(s), device(s), or technical data; or ject to export control requirements. (xii) Evidence of the reputation of the for- (3) An anti-friction bearing or bear- eign item including, if possible, information ing system not incorporated in a seg- on maintenance, repair, performance, and ment of a machine prior to shipment, other pertinent factors. but shipped as a component of a com- plete unassembled (knocked-down) ma- SUPPLEMENT NO. -
Transition Metal-Catalyzed Directed C(Sp3)–H Functionalization of Saturated Heterocycles
Synthesis Review / Short Review Transition Metal-Catalyzed Directed C(sp3)–H Functionalization of Saturated Heterocycles Daniele Antermitea James A. Bull*a a Department of Chemistry, Imperial College London, White City, Wood Lane, London, W12 0BZ, United Kingdom [email protected] Click here to insert a dedication. Received: biological interactions or selectivity profiles. The ready Accepted: Published online: availability of simple saturated heterocycle derivatives, DOI: including enantioenriched derivatives, makes them ideal Abstract Synthetic methods that can readily access saturated heterocycles starting points for further reactions. Therefore, approaches to with different substitution patterns and with control of stereo- and functionalize existing C–H bonds of these readily available regiochemistry are of huge potential value in the development of new medicinal compounds. Directed C–H functionalization of simple and building blocks appears to be of considerable potential. commercially available precursors offers the potential to prepare diverse Over the last 20 years, the concept of transition metal- collections of such valuable compounds that can probe the different available exit vectors from a ring system. Nonetheless, the presence of the catalyzed C–H functionalization has emerged with enormous Lewis basic heteroatoms makes this a significant challenge. This review potential to streamline the synthesis of complex molecules.5 covers recent advances in the catalytic C–H functionalization of saturated Specifically, transition metal catalysts can activate C–H bonds heterocycles, with a view to different heterocycles (N, O, S), substitution to form discrete C–M bonds, via different mechanistic patterns and transformations. 1. Introduction pathways.6 The resulting organometallic intermediate can then 2 a-C–H Functionalization with directing group on nitrogen form new C–C or C–heteroatom bonds with various coupling 3 C–H Functionalization at unactivated C(3), C(4) and C(5) positions partners. -
Ketoreductases Ketoreduktasen Céto-Réductases
(19) TZZ¥_¥__T (11) EP 3 134 519 B1 (12) EUROPEAN PATENT SPECIFICATION (45) Date of publication and mention (51) Int Cl.: of the grant of the patent: C12N 9/04 (2006.01) 06.06.2018 Bulletin 2018/23 (86) International application number: (21) Application number: 15717166.1 PCT/EP2015/058411 (22) Date of filing: 17.04.2015 (87) International publication number: WO 2015/162064 (29.10.2015 Gazette 2015/43) (54) KETOREDUCTASES KETOREDUKTASEN CÉTO-RÉDUCTASES (84) Designated Contracting States: • PETRI, Andreas AL AT BE BG CH CY CZ DE DK EE ES FI FR GB 04155 Leipzig (DE) GR HR HU IE IS IT LI LT LU LV MC MK MT NL NO • SCHWARZE, Daniel PL PT RO RS SE SI SK SM TR 07749 Jena (DE) • STRUHALLA, Marc (30) Priority: 22.04.2014 EP 14165444 04229 Leipzig (DE) • GREINER-STÖFFELE, Thomas (43) Date of publication of application: 99610 Sömmerda (DE) 01.03.2017 Bulletin 2017/09 (74) Representative: Kutzenberger Wolff & Partner (73) Proprietor: c-LEcta GmbH Theodor-Heuss-Ring 23 04103 Leipzig (DE) 50668 Köln (DE) (72) Inventors: (56) References cited: • SCHMIEDEL, Ramona EP-A1- 1 553 170 04277 Leipzig (DE) • VOGEL, Andreas • DATABASE PROTEIN [Online] 22 October 2013 04105 Leipzig (DE) (2013-10-22), "Short-chain dehydrogenase • KÖPKE, Sabrina [Glaciibacter superstes]", XP002731083, 04315 Leipzig (DE) retrieved from NCBI Database accession no. •CZAJA,Rico WP_022887115 04155 Leipzig (DE) • FELLER, Claudia Remarks: 04155 Leipzig (DE) Thefile contains technical information submitted after • MERKENS, Hedda the application was filed and not included in this 22395 Hamburg (DE) specification • RZEZNICKA, Kamila 04105 Leipzig (DE) Note: Within nine months of the publication of the mention of the grant of the European patent in the European Patent Bulletin, any person may give notice to the European Patent Office of opposition to that patent, in accordance with the Implementing Regulations. -
Synthesis and Polymerizability of Atom-Bridged Bicyclic Monomers
Polymers 2012, 4, 1674-1686; doi:10.3390/polym4041674 OPEN ACCESS polymers ISSN 2073-4360 www.mdpi.com/journal/polymers Review Synthesis and Polymerizability of Atom-Bridged Bicyclic Monomers Henry K. Hall, Jr. Department of Chemistry and Biochemistry, University of Arizona, 1306 E University Blvd, Tucson, AZ 85721, USA; E-Mail: [email protected]; Tel.: +1-520-621-6326; Fax: +1-520-621-8407 Received: 11 September 2012; in revised form: 19 November 2012 / Accepted: 20 November 2012 / Published: 5 December 2012 Abstract: The synthesis and polymerizability of atom-bridged bicyclic monomers was surveyed. The monomers included lactams, ureas, urethanes, lactones, carbonates, ethers, acetals, orthoesters, and amines. Despite widely-varying structures, they almost all polymerized to give polymers with monocyclic rings in the chain. The polymerizations are grouped by mechanism: uncoordinated anionic, coordinated anionic, and cationic. Keywords: alicyclic ring-containing polymers; anti-Bredt monomers; atom-bridged bicyclic monomers; ring-opening polymerizations 1. Introduction Ring-opening polymerizations, which convert cyclic monomers into linear polymers, are a major type of polymerization. A recent authoritative treatise [1] covered ring-opening polymerization of monocyclic monomers; this Review covers ring-opening polymerization of atom-bridged bicyclic monomers. “Atom-bridged” means all three chains connecting the bridgehead atoms contain at least one atom. Bicyclic compounds with bridgehead atoms directly attached to one another will not be considered here because they usually polymerize like monocyclics. Alkene metathesis of bicyclic monomers has been well-reviewed elsewhere and will not be included here. The reactions are arranged below according to mechanism: UNCOORDINATED ANIONIC POLYMERIZATIONS of lactams, ureas, and urethanes COORDINATED ANIONIC POLYMERIZATIONS of lactones and carbonates CATIONIC POLYMERIZATIONS of ethers, acetals, orthoesters and amines Polymers 2012, 4 1675 Although these monomers may appear exotic, they are often synthesized rather easily. -
Ring-Closing Metathesis for the Synthesis of Carbocyclic and Heterocyclic Intramolecular Baylis-Hillman Adducts Eunho Song
Florida State University Libraries Electronic Theses, Treatises and Dissertations The Graduate School 2005 Ring-Closing Metathesis for the Synthesis of Carbocyclic and Heterocyclic Intramolecular Baylis-Hillman Adducts Eunho Song Follow this and additional works at the FSU Digital Library. For more information, please contact [email protected] THE FLORIDA STATE UNIVERSITY COLLEGE OF ARTS AND SCIENCES RING-CLOSING METATHESIS FOR THE SYNTHESIS OF CARBOCYCLIC AND HETEROCYCLIC INTRAMOLECULAR BAYLIS-HILLMAN ADDUCTS By EUNHO SONG A Thesis submitted to the Department of Chemistry and Biochemistry in partial fulfillment of the requirements for the degree of Master of Science Degree awarded: Summer semester, 2005 The members of the Committee approve the thesis of EUNHO SONG defended on April 26, 2005. Marie E. Krafft Professor Directing Thesis Armen Zakarian Committee Member Gregory B. Dudley Committee Member Joseph B. Schlenoff Committee Member The Office of Graduate Studies has verified and approved the above named committee members. TABLE OF CONTENTS List of Tables v List of Figures vi List of Schemes xi List of Abbreviations xii Abstract xv I. INTRODUCTION Baylis-Hillman Reaction 1 Proposed Mechanism 2 Amine-Catalyzed Baylis-Hillman Reaction 3 Intramolecular Baylis-Hillman Reaction 5 Ring-Closing Metathesis Reaction 6 Proposed Mechanism 7 II. RESULTS AND DISCUSSION Optimization of reaction conditions for Baylis-Hillman Reaction 14 Synthesis of Carbocyclic RCM Products from Baylis-Hillman Adducts 16 Synthesis of Fused ring RCM Products from Baylis-Hillman Adducts 22 Synthesis of 6-Membered Carbocyclic RCM Products from Baylis 25 -Hillman Adducts using substituted hexenal Synthesis of 6-Membered Heterocyclic RCM Products from Baylis 27 -Hillman Adducts using Chiral-Amino acids 29 Summary iii III. -
Category 1—Page 1
Commerce Control List Supplement No. 1 to Part 774 Category 1—page 1 CATEGORY 1 - SPECIAL MATERIALS AND to the ITAR” (see 22 CFR parts 120 through RELATED EQUIPMENT, CHEMICALS, 130, including USML Category XXI). (2) “MICROORGANISMS,” AND “TOXINS” See also 1C009. Related Definitions: N/A Note: The Food and Drug Administration Items: (FDA) and the Drug Enforcement Administration (DEA) may control exports of items subject to the a. Seals, gaskets, sealants or fuel bladders, EAR and on the Commerce Control List. BIS “specially designed” for “aircraft” or aerospace provides cross references to these other agency use, made from more than 50% by weight of any controls for convenience only. Therefore, please of the materials controlled by 1C009.b or consult relevant FDA and DEA regulations for 1C009.c; guidance related to the item you wish to export and do not rely solely on the EAR for information b. [Reserved] about other agency export control requirements. See Supplement No. 3 to part 730 (Other U.S. Government Departments and Agencies with 1A002 “Composite” structures or laminates, Export Control Responsibilities) for as follows (see List of Items Controlled). more information. License Requirements A. “END ITEMS,” “EQUIPMENT,” Reason for Control: NS, NP, AT “ACCESSORIES,” “ATTACHMENTS,” “PARTS,” “COMPONENTS,” AND Control(s) Country Chart “SYSTEMS” (See Supp. No. 1 to part 738) 1A001 “Parts” and “components” made from NS applies to entire entry NS Column 2 fluorinated compounds, as follows (see List of NP applies to 1A002.b.1 in NP Column 1 Items Controlled). the form of tubes with an inside diameter between 75 License Requirements mm and 400 mm AT applies to entire entry AT Column 1 Reason for Control: NS, AT Reporting Requirements Country Chart Control(s) (See Supp. -
Preparatory Problems Icho 2011
rdrdrd 44434333 PREPARATORY PROBLEMS Edited by Anton Sirota 30 theoretical problems 7 practical problems 2011 THE PREPARATORY PROBLEMS FROM THE INTERNATIONAL CHEMISTRY OLYMPIADS, Series 1 The preparatory problems from the 43 rd IChO Edited by Anton Sirota IChO International Information Centre, Bratislava, Slovakia ISBN 978-80-8072-167-1 Copyright © 2016 by IUVENTA You are free to copy, distribute, transmit or adapt this publication or its parts for unlimited teaching purposes, however, you are obliged to attribute your copies, transmissions or adaptations with a reference to "The Preparatory Problems from the International Chemistry Olympiads, Series 1" as it is required in the chemical literature. The above conditions can be waived if you get permission from the copyright holder. Issued by IUVENTA in 2016 with the financial support of the Ministry of Education of the Slovak Republic Number of copies: 200 Not for sale. International Chemistry Olympiad International Information Centre IUVENTA Karloveská 64 84258 Bratislava 1, Slovakia Phone: +421-907-473367 E-mail: [email protected] Web: www.icho.sk Original title page: Preparatory Problems rd 43 International Chemistry Olympiad Editor: Saim Özkar Department of Chemistry, Middle East Technical University January 2011 Ankara Contributing Authors O. Yavuz Ataman Sezer Aygün Metin Balcı Özdemir Do ğan Jale Hacalo ğlu Hüseyin Đş çi Ahmet M. Önal Đlker Özkan Saim Özkar Cihangir Tanyeli Department of Chemistry, Middle East Technical University, 06531 Ankara, Turkey. P r e f a c e written by editor Saim Özkar (Ankara) (a shortened version) We have provided this set of problems with the intention of making the preparation for the 43 rd International Chemistry Olympiad easier for both students and mentors. -
An Overview of the Synthetic Routes to the Best Selling Drugs Containing 6-Membered Heterocycles
An overview of the synthetic routes to the best selling drugs containing 6-membered heterocycles Marcus Baumann* and Ian R. Baxendale* Review Open Access Address: Beilstein J. Org. Chem. 2013, 9, 2265–2319. Department of Chemistry, University of Durham, South Road, doi:10.3762/bjoc.9.265 Durham, DH1 3LE, UK Received: 12 July 2013 Email: Accepted: 09 October 2013 Marcus Baumann* - [email protected]; Published: 30 October 2013 Ian R. Baxendale* - [email protected] Associate Editor: P. R. Hanson * Corresponding author © 2013 Baumann and Baxendale; licensee Beilstein-Institut. Keywords: License and terms: see end of document. heterocycles; medicinal chemistry; pharmaceuticals; six-membered rings; synthesis Abstract This review which is the second in this series summarises the most common synthetic routes as applied to the preparation of many modern pharmaceutical compounds categorised as containing a six-membered heterocyclic ring. The reported examples are based on the top retailing drug molecules combining synthetic information from both scientific journals and the wider patent literature. It is hoped that this compilation, in combination with the previously published review on five-membered rings, will form a compre- hensive foundation and reference source for individuals interested in medicinal, synthetic and preparative chemistry. Introduction The commonality of six-membered heterocyclic rings in phar- Synthetic chemistry can rightfully be considered a prerequisite maceutical actives inevitably generates a substantial body of of our modern society [1]. This discipline supplies many valu- reference material. Consequently this review has been broadly able resources to our world enabling us to produce the quan- partitioned into six consecutive sections categorised by analo- tities of fertilizer needed to feed a growing world’s population gous groupings of heterocyclic rings. -
1 Heteroatom Substitution at Amide Nitrogen — Resonance Reduction and HERON 2 Reactions of Anomeric Amides 3 4 Stephen A
Preprints (www.preprints.org) | NOT PEER-REVIEWED | Posted: 8 October 2018 doi:10.20944/preprints201810.0128.v1 Peer-reviewed version available at Molecules 2018, 23, 2834; doi:10.3390/molecules23112834 1 Heteroatom Substitution at Amide Nitrogen — Resonance Reduction and HERON 2 Reactions of Anomeric Amides 3 4 Stephen A. Glover and Adam A. Rosser 5 Department of Chemistry, 6 School of Science and Technology, 7 University of New England, Armidale, NSW 2351, Australia 8 Corresponding author, Email: [email protected] 9 10 Abstract: This review describes how resonance in amides is greatly affected upon substitution 11 at nitrogen by two electronegative atoms. Nitrogen becomes strongly pyramidal and resonance 12 stabilisation, evaluated computationally, can be reduced to as little as 50% that of N,N- 13 dimethylacetamide. However, this occurs without significant twisting about the amide bond, 14 which is borne out both experimentally and theoretically. In certain configurations, reduced 15 resonance and pronounced anomeric effects between heteroatom substituents are instrumental 16 in driving the HERON (Heteroatom Rearrangement On Nitrogen)† reaction, in which the more 17 electronegative atom migrates from nitrogen to the carbonyl carbon in concert with heterolysis 18 of the amide bond, to generate acyl derivatives and heteroatom-substituted nitrenes. In other 19 cases the anomeric effect facilitates SN1 and SN2 reactivity at the amide nitrogen. 20 21 1. Introduction 22 Amides are prevalent in a range of molecules such as peptides, proteins, lactams and many 23 synthetic polymers [1]. Generically, they are composed of both a carbonyl and an amino 24 functional group, joined by a single bond between the carbon and nitrogen. -
Improved Methodology for the Preparation of Chiral Amines
Improved Methodology for the Preparation of Chiral Amines (Important Chiral Building Blocks in Pharmaceutical Drugs and Natural Products Synthesis) Mohamed Mahmoud El-Shazly A thesis submitted in partial fulfillment of the requirements for the degree of Doctor of Philosophy in Organic Synthetic Chemistry Approved Thesis Committee Prof. Dr. Thomas Nugent Professor of Organic Chemistry Jacobs University Bremen Prof. Dr. Nikolai Kuhnert Professor of Organic Chemistry Jacobs University Bremen Dr. Pralhad Ganeshpure Indian Petrochemicals Corporation Limited, India Date of Defense: August 03, 2009 School of Engineering and Science, Jacobs University, Bremen, Germany. Declaration I herewith declare that this thesis is my own work and that I have used only the sources listed. No part of this thesis has been accepted or is currently being submitted for the conferral of any degree at this university or elsewhere. Mohamed El-Shazly Bremen This dissertation is dedicated to all those people who have always given me the love, trust, and support to come to this stage of my life -To My Family- Abstract The importance of α-chiral amines as building blocks in pharmaceutical drugs, natural products, fine chemicals and agrochemicals have encouraged scientists to develop different methodologies for their preparation. Their main goal was to develop a step wise efficient and low waste production methodology which utilizes inexpensive starting material for the synthesis of α-chiral amines in high yields and enantioselectivity. Different methodologies have been developed aiming to meet these criteria. These strategies are discussed and their importance and limitations are critically analyzed. Reductive amination is a powerful methodology for the synthesis of chiral amines in high yields and enantioselectivity. -
The Most Reactive Amide As a Transition-State Mimic for Cis−Trans Interconversion † ‡ § † ∥ ⊥ Igor V
This is an open access article published under a Creative Commons Attribution (CC-BY) License, which permits unrestricted use, distribution and reproduction in any medium, provided the author and source are cited. Article pubs.acs.org/JACS The Most Reactive Amide As a Transition-State Mimic For cis−trans Interconversion † ‡ § † ∥ ⊥ Igor V. Komarov,*, Stanislav Yanik, , Aleksandr Yu. Ishchenko, , John E. Davies, ⊥ ⊥ Jonathan M. Goodman, and Anthony J. Kirby*, † ‡ Institute of High Technologies and Chemistry Department, Taras Shevchenko National University of Kyiv, Volodymyrska 60 and 64, 01601 Kyiv, Ukraine ∥ Enamine Ltd., Aleksandra Matrosova 23, 01103 Kyiv, Ukraine ⊥ University Chemical Laboratory, University of Cambridge, Cambridge CB2 1EW, United Kingdom *S Supporting Information ABSTRACT: 1-Azatricyclo[3.3.1.13,7]decan-2-one (3), the parent compound of a rare class of 90°-twisted amides, has finally been synthesized, using an unprecedented transformation. These compounds are of special interest as transition-state mimics for the enzyme-catalyzed cis−trans rotamer interconversion of amides involved in peptide and protein folding and function. The stabilization of the amide group in its high energy, perpendicular conformation common to both systems is shown for the rigid tricyclic system to depend, as predicted by calculation, on its methyl group substitution pattern, making 3 by some way the most reactive known “amide”. ■ INTRODUCTION Our current understanding of the mechanisms of bio- There is a compelling body of evidence that the cis−trans chemical transformations of this sort relies in large part upon rotamer interconversion of amide bonds in peptides and detailed knowledge of the structure and properties of reaction proteins plays a fundamental role in countless biochemical intermediates and TSs in simpler systems. -
Ref.547.2 WAN
3632 SUBJECT INDEX 1,3-dipole, 916, 917,1217, 1504, 1505 2-nitroaniline, 142, 1868 1,3-oxazine, 1913 2-nitrobenzaldehyde, 136 1,3,2-benzothiazathiolium chloride, 1395 2-nitropropane, 1336, 1337 1,3,5-trihydroxybenzene, 1498 2-nonyne, 317 1,4-benzohydroquinone, 1477 2-oxazoline, 2529 1,4-benzoquinone, 1477, 123,2042,2044 2-picoline, 2998 1,4-dimethoxybenzene, 1128 2-pyridinealdoxime, 2734 1,4-dioxygenation, 1675 2-pyrroline, 2529 1,4-hydroquinone, 1055, 1477, 1841 2-tetrahydroxybutyl-quinoxaline, 2084, 2086 1,4-naphthoquinonedibenzene-sulfonimide, 2-thiohydantoin, 2488, 2489, 2490, 2858 2147 2-vinylbutadiene, 1014 1,4-pentadiene, 1519,2881 2-vinyloxetane, 308 1,5-cyclooctadiene,803 2-vinyloxirane, 308 1,6-naphthyridine, 2335 2-vinylphosphirane,2881 1,7-dihydroxynaphthalene, 553 2,2-diethoxypropane, 200 1 1,8-naphthalenediamine,2198 2,2,4,4-tetramethylpiperidine,3146 1,8-naphthyridine, 1138 2,2' -dibenzoyloxyacetophenone, 170 IO-ketodocosanoic acid, 422 2,3,5-triphenylimidazole, 2293 l O-phenanthroline, 1572 2,3,5,6-tetramethylacetophenone, 130 12-ketoergostane, 1857 2,4-dialkoxypyrimidine, 1408 12-ketopregnane, 1857 2,4-dimethylquinoline, 1139 17-hydroxy-20-ketosteroid,877 2,4-dinitrophenylacetone, 2017 17-keto-steroid,3158 2,4-dinitrophenylhydrazine, 1007, 1086, 1633, 17-oxo-steroid, 3158 1847, 1855 18-crown-6, 704, 737, 743, 1250, 1485, 1487, 2,4-dinitrotoluene, 1381, 1895 2008,2550,2782,2848,2976 2,4,6-trinitrotoluene, 1041 IH-l,2,3-triazole,905 2,5-dimethoxytetrahydrofuran, 667, 2415, 2416 2-acetidinethione, 1222 2,6-dinitroisovanillin,