Role of Extracellular Matrix in Development and Cancer Progression
Total Page:16
File Type:pdf, Size:1020Kb
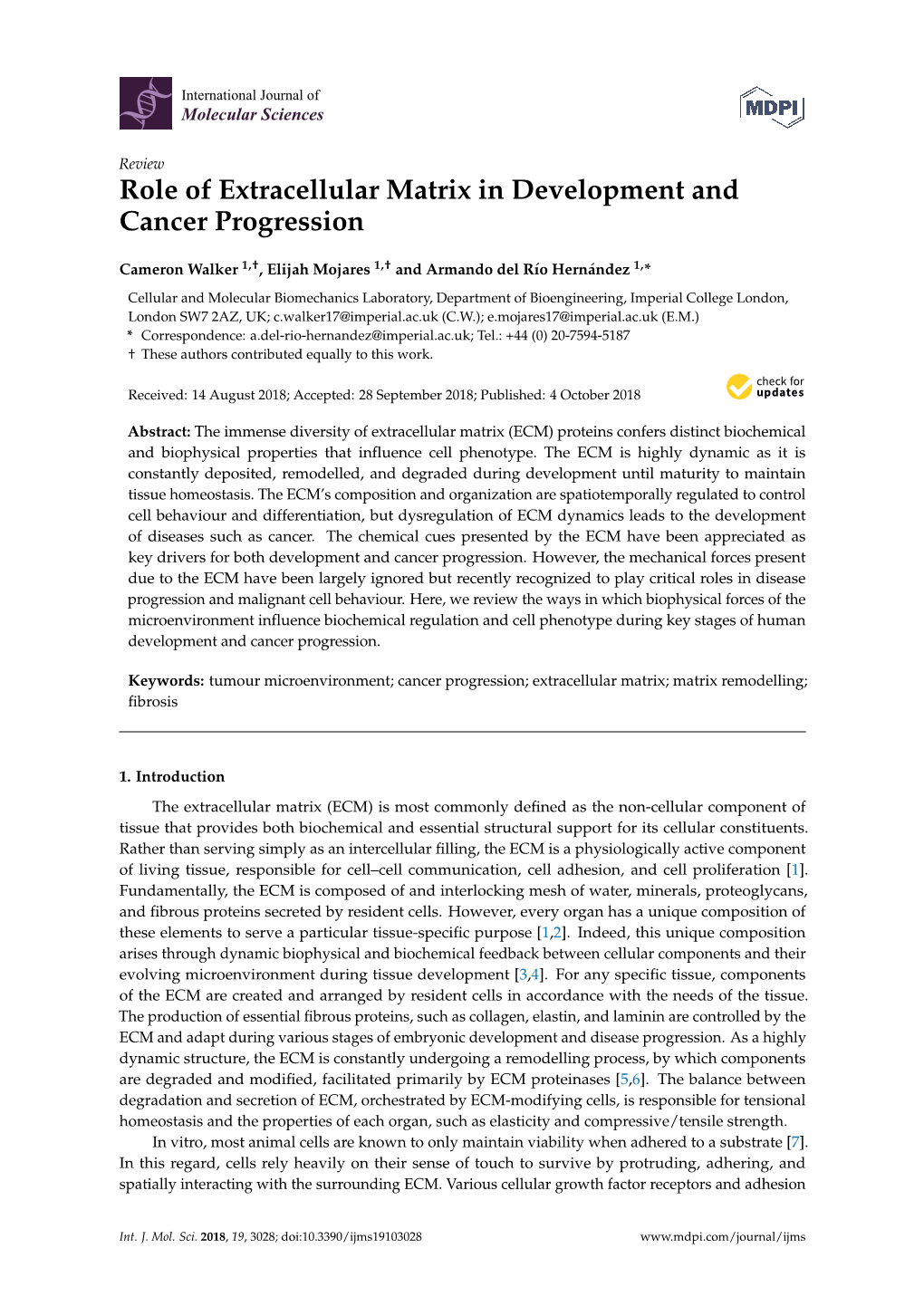
Load more
Recommended publications
-
And MMP-Mediated Cell–Matrix Interactions in the Tumor Microenvironment
International Journal of Molecular Sciences Review Hold on or Cut? Integrin- and MMP-Mediated Cell–Matrix Interactions in the Tumor Microenvironment Stephan Niland and Johannes A. Eble * Institute of Physiological Chemistry and Pathobiochemistry, University of Münster, 48149 Münster, Germany; [email protected] * Correspondence: [email protected] Abstract: The tumor microenvironment (TME) has become the focus of interest in cancer research and treatment. It includes the extracellular matrix (ECM) and ECM-modifying enzymes that are secreted by cancer and neighboring cells. The ECM serves both to anchor the tumor cells embedded in it and as a means of communication between the various cellular and non-cellular components of the TME. The cells of the TME modify their surrounding cancer-characteristic ECM. This in turn provides feedback to them via cellular receptors, thereby regulating, together with cytokines and exosomes, differentiation processes as well as tumor progression and spread. Matrix remodeling is accomplished by altering the repertoire of ECM components and by biophysical changes in stiffness and tension caused by ECM-crosslinking and ECM-degrading enzymes, in particular matrix metalloproteinases (MMPs). These can degrade ECM barriers or, by partial proteolysis, release soluble ECM fragments called matrikines, which influence cells inside and outside the TME. This review examines the changes in the ECM of the TME and the interaction between cells and the ECM, with a particular focus on MMPs. Keywords: tumor microenvironment; extracellular matrix; integrins; matrix metalloproteinases; matrikines Citation: Niland, S.; Eble, J.A. Hold on or Cut? Integrin- and MMP-Mediated Cell–Matrix 1. Introduction Interactions in the Tumor Microenvironment. -
Bruch's Membrane Abnormalities in PRDM5-Related Brittle Cornea
Porter et al. Orphanet Journal of Rare Diseases (2015) 10:145 DOI 10.1186/s13023-015-0360-4 RESEARCH Open Access Bruch’s membrane abnormalities in PRDM5-related brittle cornea syndrome Louise F. Porter1,2,3, Roberto Gallego-Pinazo4, Catherine L. Keeling5, Martyna Kamieniorz5, Nicoletta Zoppi6, Marina Colombi6, Cecilia Giunta7, Richard Bonshek2,8, Forbes D. Manson1 and Graeme C. Black1,9* Abstract Background: Brittle cornea syndrome (BCS) is a rare, generalized connective tissue disorder associated with extreme corneal thinning and a high risk of corneal rupture. Recessive mutations in transcription factors ZNF469 and PRDM5 cause BCS. Both transcription factors are suggested to act on a common pathway regulating extracellular matrix genes, particularly fibrillar collagens. We identified bilateral myopic choroidal neovascularization as the presenting feature of BCS in a 26-year-old-woman carrying a novel PRDM5 mutation (p.Glu134*). We performed immunohistochemistry of anterior and posterior segment ocular tissues, as expression of PRDM5 in the eye has not been described, or the effects of PRDM5-associated disease on the retina, particularly the extracellular matrix composition of Bruch’smembrane. Methods: Immunohistochemistry using antibodies against PRDM5, collagens type I, III, and IV was performed on the eyes of two unaffected controls and two patients (both with Δ9-14 PRDM5). Expression of collagens, integrins, tenascin and fibronectin in skin fibroblasts of a BCS patient with a novel p.Glu134* PRDM5 mutation was assessed using immunofluorescence. Results: PRDM5 is expressed in the corneal epithelium and retina. We observe reduced expression of major components of Bruch’s membrane in the eyes of two BCS patients with a PRDM5 Δ9-14 mutation. -
Collagen and Elastin Fibres
J Clin Pathol: first published as 10.1136/jcp.s3-12.1.49 on 1 January 1978. Downloaded from J. clin. Path., 31, Suppl. (Roy. Coll. Path.), 12, 49-58 Collagen and elastin fibres A. J. BAILEY From the Agricultural Research Council, Meat Research Institute, Langford, Bristol Although an understanding of the intracellular native collagen was generated from type I pro- biosynthesis of both collagen and elastin is of collagen. Whether this means that the two pro- considerable importance it is the subsequent extra- collagens are converted by different enzyme systems cellular changes involving fibrogenesis and cross- and the type III enzyme was deficient in these linking that ensure that these proteins ultimately fibroblast cultures, or that the processing of pro become the major supporting tissues of the body. type III is extremely slow, is not known. The latter This paper summarises the formation and stability proposal is consistent with the higher proportion of collagen and elastin fibres. of soluble pro type III extractable from tissue (Lenaers and Lapiere, 1975; Timpl et al., 1975). Collagen Basement membrane collagens, on the other hand, do not form fibres and this property may be The non-helical regions at the ends of the triple due to the retention of the non-helical extension helix of procollagen probably provide a number of peptides (Kefalides, 1973). In-vivo biosynthetic different intracellular functions-that is, initiating studies showing the absence of any extension peptide rapid formation of the triple helix; inhibiting intra- removal support this (Minor et al., 1976), but other cellular fibrillogenesis; and facilitating transmem- workers have reported that there is some cleavage brane movement. -
Evaluation of Elastin/Collagen Content in Human Dermis In-Vivo by Multiphoton Tomography—Variation with Depth and Correlation with Aging
Cosmetics 2014, 1, 211-221; doi:10.3390/cosmetics1030211 OPEN ACCESS cosmetics ISSN 2079-9284 www.mdpi.com/journal/cosmetics Article Evaluation of Elastin/Collagen Content in Human Dermis in-Vivo by Multiphoton Tomography—Variation with Depth and Correlation with Aging Jean-Christophe Pittet 1,*, Olga Freis 2,†, Marie-Danielle Vazquez-Duchêne 2,†, Gilles Périé 2,† and Gilles Pauly 2,† 1 Orion Concept, 100 Rue de Suède, 37100 Tours, France 2 BASF Beauty Care Solutions France SAS, 3 Rue de Seichamps, CS 71040 Pulnoy, 54272 Essey-lès-Nancy Cedex, France; E-Mails: [email protected] (O.F.); [email protected] (M.-D.V.-D.); [email protected] (G.Pé.); [email protected] (G.Pa.) † These authors contributed equally to this work. * Author to whom correspondence should be addressed; E-Mail: [email protected]; Tel.: +33-247-052-316; Fax: +33-610-786-695. Received: 14 March 2014; in revised form: 31 July 2014 / Accepted: 1 August 2014 / Published: 20 August 2014 Abstract: The aim of this study was to evaluate the influence of the depth of the dermis on the measured collagen and elastin levels and to establish the correlation between the amount of these two extracellular matrix (ECM) components and age. Multiphoton Microscopy (MPM) that measures the autofluorescence (AF) and second harmonic generation (SHG) was used to quantify the levels of elastin and collagen and to determine the SAAID (SHG-to-AF Aging Index of Dermis) at two different skin depths. A 50 MHz ultrasound scanner was used for the calculation of the Sub Epidermal Non Echogenic Band (SENEB). -
Metadherin Functions As a Laminin Receptor That Is Essential for Metastasis and Is Associated with Poor Survival in Osteosarcoma
The Texas Medical Center Library DigitalCommons@TMC The University of Texas MD Anderson Cancer Center UTHealth Graduate School of The University of Texas MD Anderson Cancer Biomedical Sciences Dissertations and Theses Center UTHealth Graduate School of (Open Access) Biomedical Sciences 5-2014 METADHERIN FUNCTIONS AS A LAMININ RECEPTOR THAT IS ESSENTIAL FOR METASTASIS AND IS ASSOCIATED WITH POOR SURVIVAL IN OSTEOSARCOMA Limin Zhu Follow this and additional works at: https://digitalcommons.library.tmc.edu/utgsbs_dissertations Part of the Cancer Biology Commons Recommended Citation Zhu, Limin, "METADHERIN FUNCTIONS AS A LAMININ RECEPTOR THAT IS ESSENTIAL FOR METASTASIS AND IS ASSOCIATED WITH POOR SURVIVAL IN OSTEOSARCOMA" (2014). The University of Texas MD Anderson Cancer Center UTHealth Graduate School of Biomedical Sciences Dissertations and Theses (Open Access). 448. https://digitalcommons.library.tmc.edu/utgsbs_dissertations/448 This Dissertation (PhD) is brought to you for free and open access by the The University of Texas MD Anderson Cancer Center UTHealth Graduate School of Biomedical Sciences at DigitalCommons@TMC. It has been accepted for inclusion in The University of Texas MD Anderson Cancer Center UTHealth Graduate School of Biomedical Sciences Dissertations and Theses (Open Access) by an authorized administrator of DigitalCommons@TMC. For more information, please contact [email protected]. METADHERIN FUNCTIONS AS A LAMININ RECEPTOR THAT IS ESSENTIAL FOR METASTASIS AND IS ASSOCIATED WITH POOR SURVIVAL IN OSTEOSARCOMA -
Vascular Phenotypes in Nonvascular Subtypes of the Ehlers-Danlos Syndrome: a Systematic Review
SYSTEMATIC REVIEW Official journal of the American College of Medical Genetics and Genomics Vascular phenotypes in nonvascular subtypes of the Ehlers-Danlos syndrome: a systematic review Sanne D’hondt, MSc1, Tim Van Damme, MD1 and Fransiska Malfait, MD, PhD1 Purpose: Within the spectrum of the Ehlers-Danlos syndromes (53%), frequently reported in musculocontractural and classical- (EDS), vascular complications are usually associated with the like EDS; intracranial hemorrhages (18%), with a high risk in vascular subtype of EDS. Vascular complications are also observed dermatosparaxis EDS; and arterial dissections (16%), frequently in other EDS subtypes, but the reports are anecdotal and the reported in kyphoscoliotic and classical EDS. Other, more minor, information is dispersed. To better document the nature of vascular vascular complications were reported in cardiac-valvular, arthro- complications among “nonvascular” EDS subtypes, we performed chalasia, spondylodysplastic, and periodontal EDS. a systematic review. Conclusion: Potentially life-threatening vascular complications are Methods: We queried three databases for English-language a rare but important finding in several nonvascular EDS sub- studies from inception until May 2017, documenting both types, highlighting a need for more systematic documentation. This phenotypes and genotypes of patients with nonvascular EDS review will help familiarize clinicians with the spectrum of vascular subtypes. The outcome included the number and nature of vascular complications in EDS and guide follow-up and management. complications. Genet Med advance online publication 5 October 2017 Results: A total of 112 papers were included and data were collected from 467 patients, of whom 77 presented with a vascular Key Words: connective tissue disorder; Ehlers-Danlos syndrome; phenotype. -
The Urokinase Receptor: a Multifunctional Receptor in Cancer Cell Biology
International Journal of Molecular Sciences Review The Urokinase Receptor: A Multifunctional Receptor in Cancer Cell Biology. Therapeutic Implications Anna Li Santi 1,†, Filomena Napolitano 2,†, Nunzia Montuori 2 and Pia Ragno 1,* 1 Department of Chemistry and Biology, University of Salerno, Fisciano, 84084 Salerno, Italy; [email protected] 2 Department of Translational Medical Sciences, “Federico II” University, 80135 Naples, Italy; fi[email protected] (F.N.); [email protected] (N.M.) * Correspondence: [email protected] † Equal contribution. Abstract: Proteolysis is a key event in several biological processes; proteolysis must be tightly con- trolled because its improper activation leads to dramatic consequences. Deregulation of proteolytic activity characterizes many pathological conditions, including cancer. The plasminogen activation (PA) system plays a key role in cancer; it includes the serine-protease urokinase-type plasminogen activator (uPA). uPA binds to a specific cellular receptor (uPAR), which concentrates proteolytic activity at the cell surface, thus supporting cell migration. However, a large body of evidence clearly showed uPAR involvement in the biology of cancer cell independently of the proteolytic activity of its ligand. In this review we will first describe this multifunctional molecule and then we will discuss how uPAR can sustain most of cancer hallmarks, which represent the biological capabilities acquired during the multistep cancer development. Finally, we will illustrate the main data available in the literature on uPAR as a cancer biomarker and a molecular target in anti-cancer therapy. Citation: Li Santi, A.; Napolitano, F.; Montuori, N.; Ragno, P. The Keywords: urokinase receptor; uPAR; cancer hallmarks Urokinase Receptor: A Multifunctional Receptor in Cancer Cell Biology. -
Reduction of Type V Collagen Using a Dominant-Negative
CORE Metadata, citation and similar papers at core.ac.uk Provided by PubMed Central Reduction of Type V Collagen Using a Dominant-negative Strategy Alters the Regulation of Fibrillogenesis and Results in the Loss of Corneal-Specific Fibril Morphology Jeffrey K. Marchant, Rita A. Hahn, Thomas F. Linsenmayer, and David E. Birk Department of Anatomy and Cellular Biology, Tufts University School of Medicine, Boston, Massachusetts 02111 Abstract. A number of factors have been implicated in synthesized the truncated or(V) protein, and this was the regulation of tissue-specific collagen fibril diameter. detectable only intracellularly, in a distribution that Previous data suggest that assembly of heterotypic colocalized with lysosomes. To assess endogenous fibrils composed of two different fibrillar collagens rep- etl(V) protein levels, infected cell cultures were as- resents a general mechanism regulating fibril diameter. sayed, and these consistently demonstrated reductions Specifically, we hypothesize that type V collagen is re- relative to control virus-infected or uninfected cultures. quired for the assembly of the small diameter fibrils ob- Analyses of corneal fibril morphology demonstrated served in the cornea. To test this, we used a dominant- that the reduction in type V collagen resulted in the as- negative retroviral strategy to decrease the levels of sembly of large-diameter fibrils with a broad size distri- type V collagen secreted by chicken corneal fibroblasts. bution, characteristics similar to fibrils produced in The chicken or(V) collagen gene was cloned, and ret- connective tissues with low type V concentrations. Im- roviral vectors that expressed a polycistronic mRNA munoelectron microscopy demonstrated the amino- encoding a truncated otl(V) minigene and the reporter terminal domain of type V collagen was associated with gene LacZ were constructed. -
Blood Vitronectin Is a Major Activator of LIF and IL-6 in the Brain Through Integrin–FAK and Upar Signaling Matthew P
© 2018. Published by The Company of Biologists Ltd | Journal of Cell Science (2018) 131, jcs202580. doi:10.1242/jcs.202580 RESEARCH ARTICLE Blood vitronectin is a major activator of LIF and IL-6 in the brain through integrin–FAK and uPAR signaling Matthew P. Keasey1, Cuihong Jia1, Lylyan F. Pimentel1,2, Richard R. Sante1, Chiharu Lovins1 and Theo Hagg1,* ABSTRACT Microglia and astrocytes express the VTN receptors αvβ3 and α β We defined how blood-derived vitronectin (VTN) rapidly and potently v 5 integrin (Herrera-Molina et al., 2012; Kang et al., 2008; activates leukemia inhibitory factor (LIF) and pro-inflammatory Milner, 2009; Welser-Alves et al., 2011). Microglia and astrocytes, interleukin 6 (IL-6) in vitro and after vascular injury in the brain. as well as endothelial cells, are major producers of pro- α in vitro Treatment with VTN (but not fibrinogen, fibronectin, laminin-111 or inflammatory cytokines, such as IL-6 and TNF , and collagen-I) substantially increased LIF and IL-6 within 4 h in after traumatic or ischemic injury to the brain (Banner et al., 1997; C6-astroglioma cells, while VTN−/− mouse plasma was less effective Erta et al., 2012; Lau and Yu, 2001) or upon self-induction by IL-6 than that from wild-type mice. LIF and IL-6 were induced by (Van Wagoner and Benveniste, 1999). IL-6 is a major regulator of a intracerebral injection of recombinant human (rh)VTN in mice, but variety of inflammatory disorders and a target for therapies (Hunter induction seen upon intracerebral hemorrhage was less in VTN−/− and Jones, 2015). -
With Caviar, Keratin & Collagen
WITH CAVIAR, KERATIN & COLLAGEN Professional treatments for colour, bleaching, care and maintenance with CAVIAR, KERATIN and COLLAGEN Professional styling products with CAVIAR, KERATIN and COLLAGEN Technical professional products with CAVIAR, KERATIN and COLLAGEN 2 Professional products by Very high technology professional products to colour, treat and protect hair from the continuous chemical and environmental stress caused on a daily basis. Formulas based on: Caviar Keratin Collagen 3 WITH CAVIAR, KERATIN & COLLAGEN Colouring permanentCream professional ammonia PPD • Respects the hair structure thanks to an exposure free free time of 12 minutes • Non-progressive • Ammonia free • Paraphenylenediamine free • Unleashes all the EFFICANCY of its active principles and maximum COLOURING POWER Mix 1 : 1 4 WITH CAVIAR, KERATIN & COLLAGEN 1. Respect for scalp and hair thanks to a shorter processing time 2. Maximum grey hair coverage 3. Lightens up to 4 tones 4. Ammonia free and Paraphenylenediamine free 5. Safe application even on customers with a sensitive scalp 6. Extreme colour brilliancy and uniformity 7. Very easy and practical to use 8. Long lasting reflections 9. High colour fastness 10. Great protection action 11. Effective restructuring action benefits 12. Maximum conditioning 5 WITH CAVIAR, KERATIN & COLLAGEN Benefits 1 2 3 Respect for scalp and hair thanks to a shorter processing Lightens up to Maximum grey hair 4 tones time coverage 6 WITH CAVIAR, KERATIN & COLLAGEN has been developed according Cosmetic colour pDT BASE Be Colour 12 Minute Dpe DIAMINOPHENOXYETHANOL to the rules of the “molar stoichiometry,” a technique creamy gel with RESORCINOL m-AMINOPHENOL of colouring clear and uncompromising. It is based on CAVIAR, a mathematical principle according to which the molar concentration of the dye base is equal to the molar KERATIN and concentration of the sum of the other colouring couplers. -
What Is Collagen?
What is Collagen? Collagen is the most abundant protein in the human body and is as diverse as it is multifunctional (1). It is found throughout the body in a variety of forms and functions making up 30 percent of your body tissue and 70 % of your skin tissue. Specifically, collagen is the protein in connective tissue that is in bone marrow, tendons, cartilage, ligaments, and linings of your body organs. It is often referred to as the glue of the body. (1) Hydrolyzed collagen means the protein has been broken down into individual amino acids which are easier for the body to absorb. Collagen serves to help repair tissue and also functions in various roles throughout the body. Collagen and the Body Locations of Collagen: What Does Collagen Do? Collagen has numerous structural properties but also plays a vital role in the repair of almost all the body’s tissues. Some diseases are directly linked to lacking this essential protein. Depending on which part of the body it is located, collagen serves different purposes. In skin: Found in the inner layer, this connective tissue gives the skin its structure and strength and also functions in the replacement of dead skin cells. A lack of collagen in the skin can contribute to a decrease in skin health leading to stretch marks, dark spots, and infections as well as affecting the skin’s ability to maintain moisture. In internal organs and blood vessels: In the lining of your organs like in the stomach, kidneys, blood vessels and spleen, collagen functions as a protective covering and a fibrous barrier. -
Understanding the Basis of Ehlers–Danlos Syndrome in the Era of the Next-Generation Sequencing
Archives of Dermatological Research (2019) 311:265–275 https://doi.org/10.1007/s00403-019-01894-0 REVIEW Understanding the basis of Ehlers–Danlos syndrome in the era of the next-generation sequencing Francesca Cortini1,2 · Chiara Villa3 · Barbara Marinelli1 · Romina Combi3 · Angela Cecilia Pesatori1 · Alessandra Bassotti4 Received: 29 July 2018 / Revised: 26 November 2018 / Accepted: 12 February 2019 / Published online: 2 March 2019 © Springer-Verlag GmbH Germany, part of Springer Nature 2019 Abstract Ehlers–Danlos syndrome (EDS) is a clinically and genetically heterogeneous group of heritable connective tissue disorders (HCTDs) defined by joint laxity, skin alterations, and joint hypermobility. The latest EDS classification recognized 13 sub- types in which the clinical and genetic phenotypes are often overlapping, making the diagnosis rather difficult and strength- ening the importance of the molecular diagnostic confirmation. New genetic techniques such as next-generation sequencing (NGS) gave the opportunity to identify the genetic bases of unresolved EDS types and support clinical counseling. To date, the molecular defects have been identified in 19 genes, mainly in those encoding collagen, its modifying enzymes or other constituents of the extracellular matrix (ECM). In this review we summarize the contribution of NGS technologies to the current knowledge of the genetic background in different EDS subtypes. Keywords Ehlers–Danlos syndrome · Heterogeneity · Heritable connective tissue disorders Introduction in 1988, represents the first attempt to classify EDS, recog- nizing 11 EDS subtypes [4], defined by Roman numerals and Ehlers–Danlos syndrome (EDS) comprises a clinically and classified according to clinical findings and the inheritance heterogeneous group of heritable connective tissue disor- pattern.