Structure-Function Studies of MICAL, the Unusual Multidomain Flavoenzyme Involved in Actin Cytoskeleton Dynamics
Total Page:16
File Type:pdf, Size:1020Kb
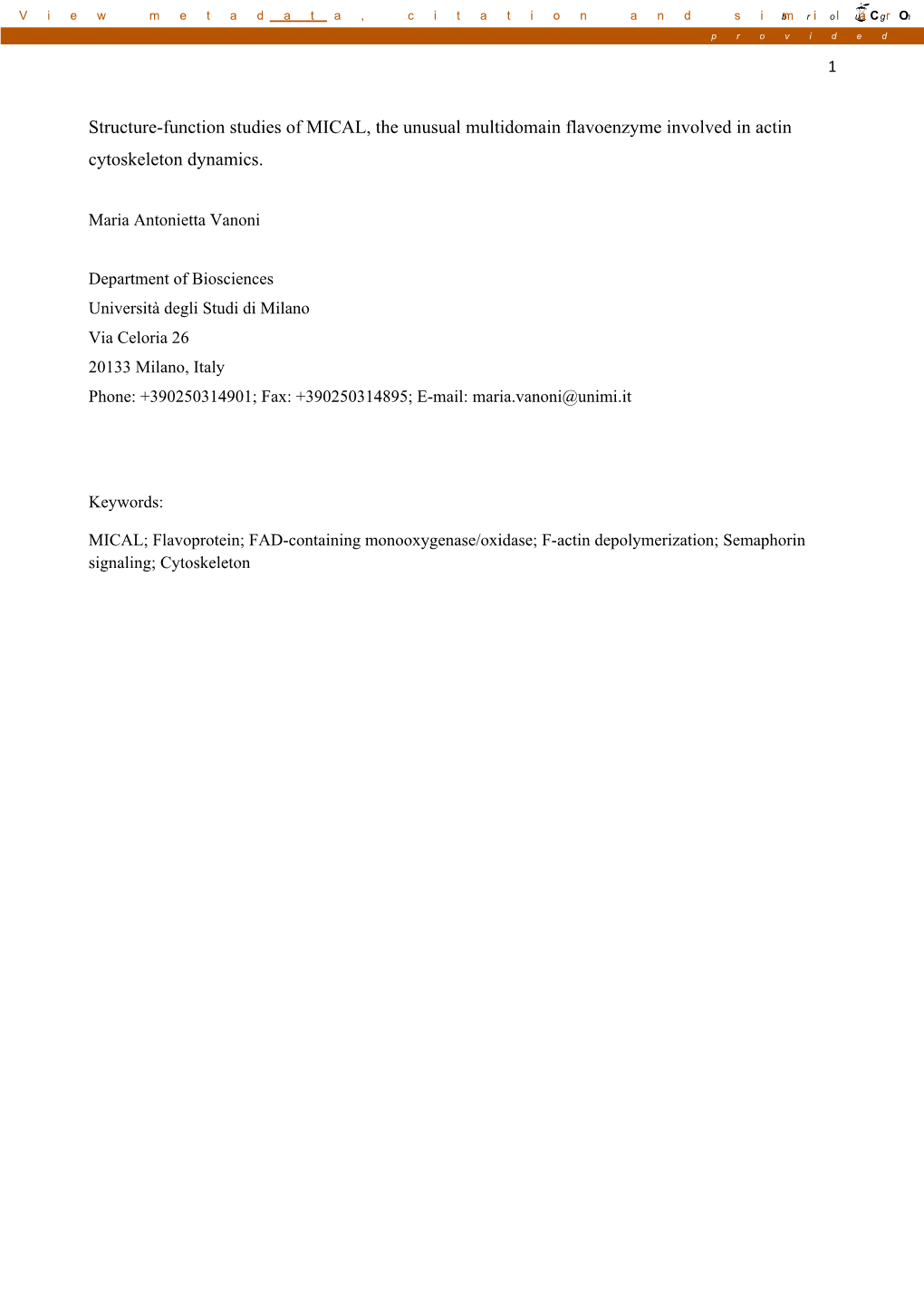
Load more
Recommended publications
-
MICAL1 Mouse Monoclonal Antibody [Clone ID: OTI6G2] Product Data
OriGene Technologies, Inc. 9620 Medical Center Drive, Ste 200 Rockville, MD 20850, US Phone: +1-888-267-4436 [email protected] EU: [email protected] CN: [email protected] Product datasheet for TA501844 MICAL1 Mouse Monoclonal Antibody [Clone ID: OTI6G2] Product data: Product Type: Primary Antibodies Clone Name: OTI6G2 Applications: FC, IF, WB Recommended Dilution: WB 1:500, IF 1:100, FLOW 1:100 Reactivity: Human Host: Mouse Isotype: IgG1 Clonality: Monoclonal Immunogen: Full length human recombinant protein of human MICAL1 (NP_073602) produced in HEK293T cell. Formulation: PBS (PH 7.3) containing 1% BSA, 50% glycerol and 0.02% sodium azide. Concentration: 1 mg/ml Purification: Purified from mouse ascites fluids or tissue culture supernatant by affinity chromatography (protein A/G) Conjugation: Unconjugated Storage: Store at -20°C as received. Stability: Stable for 12 months from date of receipt. Predicted Protein Size: 117.7 kDa Gene Name: microtubule associated monooxygenase, calponin and LIM domain containing 1 Database Link: NP_073602 Entrez Gene 64780 Human Q8TDZ2 Background: May be a cytoskeletal regulator that connects NEDD9 to intermediate filaments Synonyms: MICAL; MICAL-1; NICAL This product is to be used for laboratory only. Not for diagnostic or therapeutic use. View online » ©2021 OriGene Technologies, Inc., 9620 Medical Center Drive, Ste 200, Rockville, MD 20850, US 1 / 2 MICAL1 Mouse Monoclonal Antibody [Clone ID: OTI6G2] – TA501844 Product images: HEK293T cells were transfected with the pCMV6- ENTRY control (Left lane) or pCMV6-ENTRY MICAL1 ([RC208308], Right lane) cDNA for 48 hrs and lysed. Equivalent amounts of cell lysates (5 ug per lane) were separated by SDS-PAGE and immunoblotted with anti-MICAL1. -
A Computational Approach for Defining a Signature of Β-Cell Golgi Stress in Diabetes Mellitus
Page 1 of 781 Diabetes A Computational Approach for Defining a Signature of β-Cell Golgi Stress in Diabetes Mellitus Robert N. Bone1,6,7, Olufunmilola Oyebamiji2, Sayali Talware2, Sharmila Selvaraj2, Preethi Krishnan3,6, Farooq Syed1,6,7, Huanmei Wu2, Carmella Evans-Molina 1,3,4,5,6,7,8* Departments of 1Pediatrics, 3Medicine, 4Anatomy, Cell Biology & Physiology, 5Biochemistry & Molecular Biology, the 6Center for Diabetes & Metabolic Diseases, and the 7Herman B. Wells Center for Pediatric Research, Indiana University School of Medicine, Indianapolis, IN 46202; 2Department of BioHealth Informatics, Indiana University-Purdue University Indianapolis, Indianapolis, IN, 46202; 8Roudebush VA Medical Center, Indianapolis, IN 46202. *Corresponding Author(s): Carmella Evans-Molina, MD, PhD ([email protected]) Indiana University School of Medicine, 635 Barnhill Drive, MS 2031A, Indianapolis, IN 46202, Telephone: (317) 274-4145, Fax (317) 274-4107 Running Title: Golgi Stress Response in Diabetes Word Count: 4358 Number of Figures: 6 Keywords: Golgi apparatus stress, Islets, β cell, Type 1 diabetes, Type 2 diabetes 1 Diabetes Publish Ahead of Print, published online August 20, 2020 Diabetes Page 2 of 781 ABSTRACT The Golgi apparatus (GA) is an important site of insulin processing and granule maturation, but whether GA organelle dysfunction and GA stress are present in the diabetic β-cell has not been tested. We utilized an informatics-based approach to develop a transcriptional signature of β-cell GA stress using existing RNA sequencing and microarray datasets generated using human islets from donors with diabetes and islets where type 1(T1D) and type 2 diabetes (T2D) had been modeled ex vivo. To narrow our results to GA-specific genes, we applied a filter set of 1,030 genes accepted as GA associated. -
Genome-Wide Rnai Screening Identifies Human Proteins with A
RESOURCES Genome-wide RNAi screening identifies human proteins with a regulatory function in the early secretory pathway Jeremy C. Simpson1,7, Brigitte Joggerst2, Vibor Laketa2, Fatima Verissimo2, Cihan Cetin2, Holger Erfle2,6, Mariana G. Bexiga1, Vasanth R. Singan1, Jean-Karim Hériché3, Beate Neumann3, Alvaro Mateos2, Jonathon Blake4, Stephanie Bechtel5, Vladimir Benes4, Stefan Wiemann5, Jan Ellenberg2,3 and Rainer Pepperkok2,7 The secretory pathway in mammalian cells has evolved to facilitate the transfer of cargo molecules to internal and cell surface membranes. Use of automated microscopy-based genome-wide RNA interference screens in cultured human cells allowed us to identify 554 proteins influencing secretion. Cloning, fluorescent-tagging and subcellular localization analysis of 179 of these proteins revealed that more than two-thirds localize to either the cytoplasm or membranes of the secretory and endocytic pathways. The depletion of 143 of them resulted in perturbations in the organization of the COPII and/or COPI vesicular coat complexes of the early secretory pathway, or the morphology of the Golgi complex. Network analyses revealed a so far unappreciated link between early secretory pathway function, small GTP-binding protein regulation, actin cytoskeleton organization and EGF-receptor-mediated signalling. This work provides an important resource for an integrative understanding of global cellular organization and regulation of the secretory pathway in mammalian cells. Within higher eukaryotic cells membrane traffic pathways connect the Extensive efforts over many years have revealed a significant number various membrane-bounded organelles, thereby ensuring that they of regulators associated with the secretory pathway. Early biochemical retain the correct complement of proteins and lipids to maintain approaches to identify individual machinery components have started cellular homeostasis. -
Aneuploidy: Using Genetic Instability to Preserve a Haploid Genome?
Health Science Campus FINAL APPROVAL OF DISSERTATION Doctor of Philosophy in Biomedical Science (Cancer Biology) Aneuploidy: Using genetic instability to preserve a haploid genome? Submitted by: Ramona Ramdath In partial fulfillment of the requirements for the degree of Doctor of Philosophy in Biomedical Science Examination Committee Signature/Date Major Advisor: David Allison, M.D., Ph.D. Academic James Trempe, Ph.D. Advisory Committee: David Giovanucci, Ph.D. Randall Ruch, Ph.D. Ronald Mellgren, Ph.D. Senior Associate Dean College of Graduate Studies Michael S. Bisesi, Ph.D. Date of Defense: April 10, 2009 Aneuploidy: Using genetic instability to preserve a haploid genome? Ramona Ramdath University of Toledo, Health Science Campus 2009 Dedication I dedicate this dissertation to my grandfather who died of lung cancer two years ago, but who always instilled in us the value and importance of education. And to my mom and sister, both of whom have been pillars of support and stimulating conversations. To my sister, Rehanna, especially- I hope this inspires you to achieve all that you want to in life, academically and otherwise. ii Acknowledgements As we go through these academic journeys, there are so many along the way that make an impact not only on our work, but on our lives as well, and I would like to say a heartfelt thank you to all of those people: My Committee members- Dr. James Trempe, Dr. David Giovanucchi, Dr. Ronald Mellgren and Dr. Randall Ruch for their guidance, suggestions, support and confidence in me. My major advisor- Dr. David Allison, for his constructive criticism and positive reinforcement. -
Pan-Cancer Analysis Identifies Mutations in SUGP1 That Recapitulate Mutant SF3B1 Splicing Dysregulation
Pan-cancer analysis identifies mutations in SUGP1 that recapitulate mutant SF3B1 splicing dysregulation Zhaoqi Liua,b,c,1, Jian Zhangd,1, Yiwei Suna,c, Tomin E. Perea-Chambleea,b,c, James L. Manleyd,2, and Raul Rabadana,b,c,2 aProgram for Mathematical Genomics, Columbia University, New York, NY 10032; bDepartment of Systems Biology, Columbia University, New York, NY 10032; cDepartment of Biomedical Informatics, Columbia University, New York, NY 10032; and dDepartment of Biological Sciences, Columbia University, New York, NY 10027 Contributed by James L. Manley, March 2, 2020 (sent for review January 2, 2020; reviewed by Kristen Lynch and Gene Yeo) The gene encoding the core spliceosomal protein SF3B1 is the most also resulted in the same splicing defects observed in SF3B1 frequently mutated gene encoding a splicing factor in a variety of mutant cells (11). hematologic malignancies and solid tumors. SF3B1 mutations in- In addition to SF3B1, other SF-encoding genes have also been duce use of cryptic 3′ splice sites (3′ss), and these splicing errors found to be mutated in hematologic malignancies, e.g., U2AF1, contribute to tumorigenesis. However, it is unclear how wide- SRSF2, and ZRSR2. However, these SF gene mutations do not spread this type of cryptic 3′ss usage is in cancers and what is share common alterations in splicing (1, 3), suggesting that dif- the full spectrum of genetic mutations that cause such missplicing. ferent splicing patterns may contribute to different phenotypes To address this issue, we performed an unbiased pan-cancer anal- of cancers. Because SF3B1 is the most frequently mutated ysis to identify genetic alterations that lead to the same aberrant splicing gene, the splicing defects caused by mutant SF3B1 may SF3B1 splicing as observed with mutations. -
CHML Promotes Liver Cancer Metastasis by Facilitating Rab14 Recycle
ARTICLE https://doi.org/10.1038/s41467-019-10364-0 OPEN CHML promotes liver cancer metastasis by facilitating Rab14 recycle Tian-Wei Chen1,2,3, Fen-Fen Yin1,2, Yan-Mei Yuan1,2, Dong-Xian Guan1, Erbin Zhang1,2, Feng-Kun Zhang1,2, Hao Jiang1,2, Ning Ma1,2, Jing-Jing Wang1, Qian-Zhi Ni1,2, Lin Qiu1, Jing Feng3, Xue-Li Zhang3, Ying Bao4, Kang Wang5, Shu-Qun Cheng5, Xiao-Fan Wang6, Xiang Wang7, Jing-Jing Li1,2 & Dong Xie1,2,8,9 Metastasis-associated recurrence is the major cause of poor prognosis in hepatocellular 1234567890():,; carcinoma (HCC), however, the underlying mechanisms remain largely elusive. In this study, we report that expression of choroideremia-like (CHML) is increased in HCC, associated with poor survival, early recurrence and more satellite nodules in HCC patients. CHML promotes migration, invasion and metastasis of HCC cells, in a Rab14-dependent manner. Mechanism study reveals that CHML facilitates constant recycling of Rab14 by escorting Rab14 to the membrane. Furthermore, we identify several metastasis regulators as cargoes carried by Rab14-positive vesicles, including Mucin13 and CD44, which may contribute to metastasis- promoting effects of CHML. Altogether, our data establish CHML as a potential promoter of HCC metastasis, and the CHML-Rab14 axis may be a promising therapeutic target for HCC. 1 CAS Key Laboratory of Nutrition, Metabolism and Food Safety, Shanghai Institute of Nutrition and Health, Shanghai Institutes for Biological Sciences, Chinese Academy of Sciences, Xuhui district 200031, China. 2 University of Chinese Academy of Sciences, Chinese Academy of Sciences, Xuhui district 200031, China. 3 Department of General Surgery, Fengxian Hospital Affiliated to Southern Medical University, 6600 Nanfeng Road, Shanghai 201499, China. -
Expression Pattern of the PRDX2, RAB1A, RAB1B, RAB5A and RAB25 Genes in Normal and Cancer Cervical Tissues
INTERNATIONAL JOURNAL OF ONCOLOGY 46: 107-112, 2015 Expression pattern of the PRDX2, RAB1A, RAB1B, RAB5A and RAB25 genes in normal and cancer cervical tissues ANDREJ NIKOSHKOV1, KRISTINA BROLIDEN2, SANAZ ATTARHA1,3, VITALI SVIATOHA3, ANN-CATHRIN HELLSTRÖM4, MIRIAM MINTS1 and SONIA ANDERSSON1 1Department of Women's and Children's Health, Division of Obstetrics and Gynecology, Karolinska Institute, Karolinska University Hospital Solna, 171 76 Stockholm; 2Department of Medicine Solna, Unit of Infectious Diseases, Center for Molecular Medicine, Karolinska Institute, Karolinska University Hospital, 171 76 Stockholm; 3Department of Oncology-Pathology, Karolinska Institute, 171 76 Stockholm; 4Department of Gynecological Oncology, Radiumhemmet, Karolinska University Hospital, 171 76 Stockholm, Sweden Received July 11, 2014; Accepted August 28, 2014 DOI: 10.3892/ijo.2014.2724 Abstract. Cervical cancer is the second most prevalent RAB1B transcript occurs in normal cervical tissue and in malignancy among women worldwide, and additional preinvasive cervical lesions; not in invasive cervical tumors. objective diagnostic markers for this disease are needed. Given the link between cancer development and alternative Introduction splicing, we aimed to analyze the splicing patterns of the PRDX2, RAB1A, RAB1B, RAB5A and RAB25 genes, which Alternative splicing is a process in which either different are associated with different cancers, in normal cervical combinations of exons or parts of exons are employed to tissue, preinvasive cervical lesions and invasive cervical generate new transcripts through the use of alternative-splicing tumors, to identify new objective diagnostic markers. Biopsies sites. More than 90% of human intron-containing genes of normal cervical tissue, preinvasive cervical lesions and undergo alternative splicing, which allows a single transcript invasive cervical tumors, were subjected to rapid amplification to encode a number of RNAs that produce various protein of cDNA 3' ends (3' RACE) RT‑PCR. -
Bioinformatics Analysis for the Identification of Differentially Expressed Genes and Related Signaling Pathways in H
Bioinformatics analysis for the identification of differentially expressed genes and related signaling pathways in H. pylori-CagA transfected gastric cancer cells Dingyu Chen*, Chao Li, Yan Zhao, Jianjiang Zhou, Qinrong Wang and Yuan Xie* Key Laboratory of Endemic and Ethnic Diseases , Ministry of Education, Guizhou Medical University, Guiyang, China * These authors contributed equally to this work. ABSTRACT Aim. Helicobacter pylori cytotoxin-associated protein A (CagA) is an important vir- ulence factor known to induce gastric cancer development. However, the cause and the underlying molecular events of CagA induction remain unclear. Here, we applied integrated bioinformatics to identify the key genes involved in the process of CagA- induced gastric epithelial cell inflammation and can ceration to comprehend the potential molecular mechanisms involved. Materials and Methods. AGS cells were transected with pcDNA3.1 and pcDNA3.1::CagA for 24 h. The transfected cells were subjected to transcriptome sequencing to obtain the expressed genes. Differentially expressed genes (DEG) with adjusted P value < 0.05, | logFC |> 2 were screened, and the R package was applied for gene ontology (GO) enrichment and the Kyoto Encyclopedia of Genes and Genomes (KEGG) pathway analysis. The differential gene protein–protein interaction (PPI) network was constructed using the STRING Cytoscape application, which conducted visual analysis to create the key function networks and identify the key genes. Next, the Submitted 20 August 2020 Kaplan–Meier plotter survival analysis tool was employed to analyze the survival of the Accepted 11 March 2021 key genes derived from the PPI network. Further analysis of the key gene expressions Published 15 April 2021 in gastric cancer and normal tissues were performed based on The Cancer Genome Corresponding author Atlas (TCGA) database and RT-qPCR verification. -
The Genetics of Bipolar Disorder
Molecular Psychiatry (2008) 13, 742–771 & 2008 Nature Publishing Group All rights reserved 1359-4184/08 $30.00 www.nature.com/mp FEATURE REVIEW The genetics of bipolar disorder: genome ‘hot regions,’ genes, new potential candidates and future directions A Serretti and L Mandelli Institute of Psychiatry, University of Bologna, Bologna, Italy Bipolar disorder (BP) is a complex disorder caused by a number of liability genes interacting with the environment. In recent years, a large number of linkage and association studies have been conducted producing an extremely large number of findings often not replicated or partially replicated. Further, results from linkage and association studies are not always easily comparable. Unfortunately, at present a comprehensive coverage of available evidence is still lacking. In the present paper, we summarized results obtained from both linkage and association studies in BP. Further, we indicated new potential interesting genes, located in genome ‘hot regions’ for BP and being expressed in the brain. We reviewed published studies on the subject till December 2007. We precisely localized regions where positive linkage has been found, by the NCBI Map viewer (http://www.ncbi.nlm.nih.gov/mapview/); further, we identified genes located in interesting areas and expressed in the brain, by the Entrez gene, Unigene databases (http://www.ncbi.nlm.nih.gov/entrez/) and Human Protein Reference Database (http://www.hprd.org); these genes could be of interest in future investigations. The review of association studies gave interesting results, as a number of genes seem to be definitively involved in BP, such as SLC6A4, TPH2, DRD4, SLC6A3, DAOA, DTNBP1, NRG1, DISC1 and BDNF. -
Rapid Diagnosis of Medulloblastoma Molecular Subgroups
Published OnlineFirst February 16, 2011; DOI: 10.1158/1078-0432.CCR-10-2210 Clinical Cancer Imaging, Diagnosis, Prognosis Research Rapid Diagnosis of Medulloblastoma Molecular Subgroups Ed C. Schwalbe1, Janet C. Lindsey1, Debbie Straughton1, Twala L. Hogg2, Michael Cole1, Hisham Megahed1, Sarra L. Ryan1, Meryl E. Lusher1, Michael D. Taylor4, Richard J. Gilbertson2, David W. Ellison3, Simon Bailey1, and Steven C. Clifford1 Abstract Purpose: Microarray studies indicate medulloblastoma comprises distinct molecular disease subgroups, which offer potential for improved clinical management. Experimental Design: Minimal mRNA expression signatures diagnostic for the Wnt/Wingless (WNT) and Sonic Hedgehog (SHH) subgroups were developed, validated, and used to assign subgroup affiliation in 173 tumors from four independent cohorts, alongside a systematic investigation of subgroup clinical and molecular characteristics. Results: WNT tumors [12% (21/173)] were diagnosed >5 years of age (peak, 10 years), displayed classic histology, CTNNB1 mutation (19/20), and associated chromosome 6 loss, and have previously been associated with favorable prognosis. SHH cases [24% (42/173)] predominated in infants (<3 years) and showed an age-dependent relationship to desmoplastic/nodular pathology; all infant desmoplastic/ nodular cases (previously associated with a good outcome) were SHH-positive, but these relationships broke down in noninfants. PTCH1 mutations were common [34% (11/32)], but PTCH1 exon1c hypermethylation, chromosome 9q and REN (KCTD11) genetic loss were not SHH associated, and SMO or SUFU mutation, PTCH1 exon1a or SUFU hypermethylation did not play a role, indicating novel activating mechanisms in the majority of SHH cases. SHH tumors were associated with an absence of COL1A2 methylation. WNT/SHH-independent medulloblastomas [64% (110/173)] showed all histolo- gies, peaked at 3 and 6 years, and were exclusively associated with chromosome 17p loss. -
Human Induced Pluripotent Stem Cell–Derived Podocytes Mature Into Vascularized Glomeruli Upon Experimental Transplantation
BASIC RESEARCH www.jasn.org Human Induced Pluripotent Stem Cell–Derived Podocytes Mature into Vascularized Glomeruli upon Experimental Transplantation † Sazia Sharmin,* Atsuhiro Taguchi,* Yusuke Kaku,* Yasuhiro Yoshimura,* Tomoko Ohmori,* ‡ † ‡ Tetsushi Sakuma, Masashi Mukoyama, Takashi Yamamoto, Hidetake Kurihara,§ and | Ryuichi Nishinakamura* *Department of Kidney Development, Institute of Molecular Embryology and Genetics, and †Department of Nephrology, Faculty of Life Sciences, Kumamoto University, Kumamoto, Japan; ‡Department of Mathematical and Life Sciences, Graduate School of Science, Hiroshima University, Hiroshima, Japan; §Division of Anatomy, Juntendo University School of Medicine, Tokyo, Japan; and |Japan Science and Technology Agency, CREST, Kumamoto, Japan ABSTRACT Glomerular podocytes express proteins, such as nephrin, that constitute the slit diaphragm, thereby contributing to the filtration process in the kidney. Glomerular development has been analyzed mainly in mice, whereas analysis of human kidney development has been minimal because of limited access to embryonic kidneys. We previously reported the induction of three-dimensional primordial glomeruli from human induced pluripotent stem (iPS) cells. Here, using transcription activator–like effector nuclease-mediated homologous recombination, we generated human iPS cell lines that express green fluorescent protein (GFP) in the NPHS1 locus, which encodes nephrin, and we show that GFP expression facilitated accurate visualization of nephrin-positive podocyte formation in -
Genomic and Transcriptome Analysis Revealing an Oncogenic Functional Module in Meningiomas
Neurosurg Focus 35 (6):E3, 2013 ©AANS, 2013 Genomic and transcriptome analysis revealing an oncogenic functional module in meningiomas XIAO CHANG, PH.D.,1 LINGLING SHI, PH.D.,2 FAN GAO, PH.D.,1 JONATHAN RUssIN, M.D.,3 LIYUN ZENG, PH.D.,1 SHUHAN HE, B.S.,3 THOMAS C. CHEN, M.D.,3 STEVEN L. GIANNOTTA, M.D.,3 DANIEL J. WEISENBERGER, PH.D.,4 GAbrIEL ZADA, M.D.,3 KAI WANG, PH.D.,1,5,6 AND WIllIAM J. MAck, M.D.1,3 1Zilkha Neurogenetic Institute, Keck School of Medicine, University of Southern California, Los Angeles, California; 2GHM Institute of CNS Regeneration, Jinan University, Guangzhou, China; 3Department of Neurosurgery, Keck School of Medicine, University of Southern California, Los Angeles, California; 4USC Epigenome Center, Keck School of Medicine, University of Southern California, Los Angeles, California; 5Department of Psychiatry, Keck School of Medicine, University of Southern California, Los Angeles, California; and 6Division of Bioinformatics, Department of Preventive Medicine, Keck School of Medicine, University of Southern California, Los Angeles, California Object. Meningiomas are among the most common primary adult brain tumors. Although typically benign, roughly 2%–5% display malignant pathological features. The key molecular pathways involved in malignant trans- formation remain to be determined. Methods. Illumina expression microarrays were used to assess gene expression levels, and Illumina single- nucleotide polymorphism arrays were used to identify copy number variants in benign, atypical, and malignant me- ningiomas (19 tumors, including 4 malignant ones). The authors also reanalyzed 2 expression data sets generated on Affymetrix microarrays (n = 68, including 6 malignant ones; n = 56, including 3 malignant ones).