Diterpene Cyclases and the Nature of the Isoprene Fold
Total Page:16
File Type:pdf, Size:1020Kb
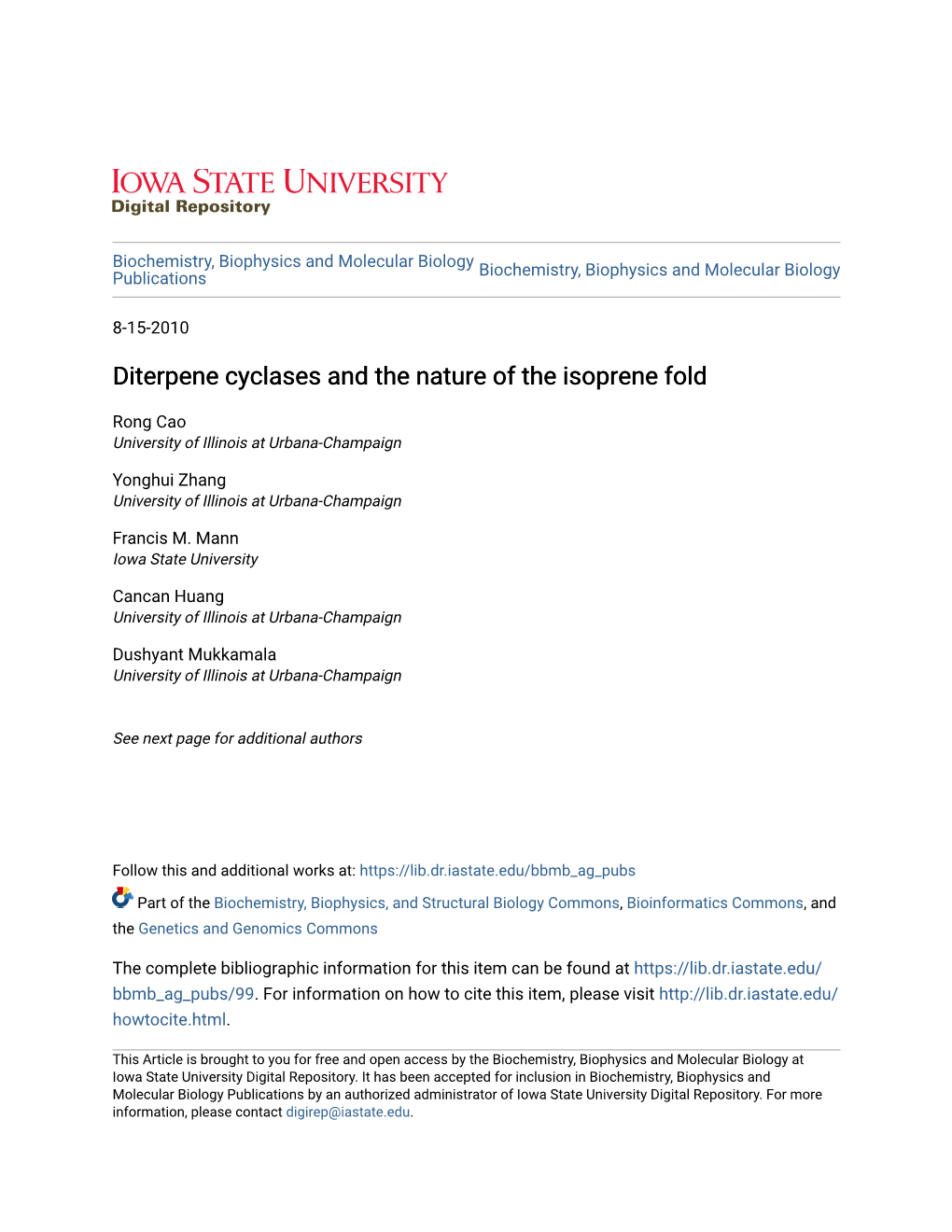
Load more
Recommended publications
-
The Phytochemistry of Cherokee Aromatic Medicinal Plants
medicines Review The Phytochemistry of Cherokee Aromatic Medicinal Plants William N. Setzer 1,2 1 Department of Chemistry, University of Alabama in Huntsville, Huntsville, AL 35899, USA; [email protected]; Tel.: +1-256-824-6519 2 Aromatic Plant Research Center, 230 N 1200 E, Suite 102, Lehi, UT 84043, USA Received: 25 October 2018; Accepted: 8 November 2018; Published: 12 November 2018 Abstract: Background: Native Americans have had a rich ethnobotanical heritage for treating diseases, ailments, and injuries. Cherokee traditional medicine has provided numerous aromatic and medicinal plants that not only were used by the Cherokee people, but were also adopted for use by European settlers in North America. Methods: The aim of this review was to examine the Cherokee ethnobotanical literature and the published phytochemical investigations on Cherokee medicinal plants and to correlate phytochemical constituents with traditional uses and biological activities. Results: Several Cherokee medicinal plants are still in use today as herbal medicines, including, for example, yarrow (Achillea millefolium), black cohosh (Cimicifuga racemosa), American ginseng (Panax quinquefolius), and blue skullcap (Scutellaria lateriflora). This review presents a summary of the traditional uses, phytochemical constituents, and biological activities of Cherokee aromatic and medicinal plants. Conclusions: The list is not complete, however, as there is still much work needed in phytochemical investigation and pharmacological evaluation of many traditional herbal medicines. Keywords: Cherokee; Native American; traditional herbal medicine; chemical constituents; pharmacology 1. Introduction Natural products have been an important source of medicinal agents throughout history and modern medicine continues to rely on traditional knowledge for treatment of human maladies [1]. Traditional medicines such as Traditional Chinese Medicine [2], Ayurvedic [3], and medicinal plants from Latin America [4] have proven to be rich resources of biologically active compounds and potential new drugs. -
Identification and Functional Characterization of Diterpene Synthases for Triptolide Biosynthesis from Tripterygium Wilfordii
Biochemistry, Biophysics and Molecular Biology Publications Biochemistry, Biophysics and Molecular Biology 1-2018 Identification and functional characterization of diterpene synthases for triptolide biosynthesis from Tripterygium wilfordii Ping Su China Academy of Chinese Medical Sciences Hongyu Guan China Academy of Chinese Medical Sciences Yujun Zhao China Academy of Chinese Medical Sciences Yuru Tong China Academy of Chinese Medical Sciences Meimei Xu Iowa State University, [email protected] See next page for additional authors Follow this and additional works at: https://lib.dr.iastate.edu/bbmb_ag_pubs Part of the Biochemistry Commons, Biophysics Commons, Molecular Biology Commons, Plant Sciences Commons, and the Structural Biology Commons The complete bibliographic information for this item can be found at https://lib.dr.iastate.edu/ bbmb_ag_pubs/272. For information on how to cite this item, please visit http://lib.dr.iastate.edu/howtocite.html. This Article is brought to you for free and open access by the Biochemistry, Biophysics and Molecular Biology at Iowa State University Digital Repository. It has been accepted for inclusion in Biochemistry, Biophysics and Molecular Biology Publications by an authorized administrator of Iowa State University Digital Repository. For more information, please contact [email protected]. Identification and functional characterization of diterpene synthases for triptolide biosynthesis from Tripterygium wilfordii Abstract Tripterygium wilfordii has long been used as a medicinal plant, and exhibits impressive and effective anti- inflammatory, immunosuppressive and anti-tumor activities. The main active ingredients are diterpenoids and triterpenoids, such as triptolide and celastrol, respectively. A major challenge to harnessing these natural products is that they are found in very low amounts in planta. -
Medically Useful Plant Terpenoids: Biosynthesis, Occurrence, and Mechanism of Action
molecules Review Medically Useful Plant Terpenoids: Biosynthesis, Occurrence, and Mechanism of Action Matthew E. Bergman 1 , Benjamin Davis 1 and Michael A. Phillips 1,2,* 1 Department of Cellular and Systems Biology, University of Toronto, Toronto, ON M5S 3G5, Canada; [email protected] (M.E.B.); [email protected] (B.D.) 2 Department of Biology, University of Toronto–Mississauga, Mississauga, ON L5L 1C6, Canada * Correspondence: [email protected]; Tel.: +1-905-569-4848 Academic Editors: Ewa Swiezewska, Liliana Surmacz and Bernhard Loll Received: 3 October 2019; Accepted: 30 October 2019; Published: 1 November 2019 Abstract: Specialized plant terpenoids have found fortuitous uses in medicine due to their evolutionary and biochemical selection for biological activity in animals. However, these highly functionalized natural products are produced through complex biosynthetic pathways for which we have a complete understanding in only a few cases. Here we review some of the most effective and promising plant terpenoids that are currently used in medicine and medical research and provide updates on their biosynthesis, natural occurrence, and mechanism of action in the body. This includes pharmacologically useful plastidic terpenoids such as p-menthane monoterpenoids, cannabinoids, paclitaxel (taxol®), and ingenol mebutate which are derived from the 2-C-methyl-d-erythritol-4-phosphate (MEP) pathway, as well as cytosolic terpenoids such as thapsigargin and artemisinin produced through the mevalonate (MVA) pathway. We further provide a review of the MEP and MVA precursor pathways which supply the carbon skeletons for the downstream transformations yielding these medically significant natural products. Keywords: isoprenoids; plant natural products; terpenoid biosynthesis; medicinal plants; terpene synthases; cytochrome P450s 1. -
A Multivariate Approach to Integration of Ethnobotanical, Pharmacological
A Multivariate Approach to Integration of Ethnobotanical, Pharmacological, and Phytochemical Analyses of Cree and Squamish Traditional Herbal Medicines for Anti-Diabetes Use Braydon Hall, BSc. A thesis submitted to the Faculty of Graduate and Postdoctoral Studies in partial fulfillment of the requirements for the M. Sc. Degree in Biology Department of Biology Faculty of Science University of Ottawa ©Braydon Hall, Ottawa, Canada, 2019 ABSTRACT This thesis investigated the integration of pharmacological and phytochemical data of medicinal plants from the Cree of Eeyou Istchee in Northern Quebec. Data from these 17 plant extracts were assessed for patterns of biological activity and chemical signals that could be explained by taxonomic or plant organ groupings. The Squamish medicinal plant Oplopanax horridus (Sm.) Miq. was also assessed for enzyme inhibition activity across multiple extracts and for bioactive compounds using an untargeted metabolomics approach. A comprehensive data set was assembled documenting the relative activities on the 17 plant extracts in 69 cell-free and cell-based bioassays covering activity on glucohomeostasis, effects of hyperglycemia, and capacity for enzyme inhibition. Multivariate analysis suggests that the leaf part extracts are particularly associated with antioxidant and antiglycation activities, while another discrete group of extracts associate strongly with other sets of glucohomeostasis assays. The activity of extracts on enzyme inhibition appears to be the factor most strongly driving the majority of activity patterns, likely because extracts that interact strongly with more metabolic enzymes will have more effects on other targets in the body. The phytochemical profiles of the Cree medicinal plants were assessed in two ways. First, spectroscopic and chromatographic data for the plant extracts was compared to a database of phytochemical standards using a proprietary Waters software, UNIFI, to match known signals of chemical standards to unidentified peaks in the plant extracts. -
Biochemistry of Terpenes and Recent Advances in Plant Protection
International Journal of Molecular Sciences Review Biochemistry of Terpenes and Recent Advances in Plant Protection Vincent Ninkuu , Lin Zhang, Jianpei Yan, Zhenchao Fu, Tengfeng Yang and Hongmei Zeng * State Key Laboratory for Biology of Plant Diseases and Insect Pests, Institute of Plant Protection, Chinese Academy of Agricultural Sciences (IPP_CAAS), Beijing 100193, China; [email protected] (V.N.); [email protected] (L.Z.); [email protected] (J.Y.); [email protected] (Z.F.); [email protected] (T.Y.) * Correspondence: [email protected]; Tel.: +86-10-82109562 Abstract: Biodiversity is adversely affected by the growing levels of synthetic chemicals released into the environment due to agricultural activities. This has been the driving force for embracing sustainable agriculture. Plant secondary metabolites offer promising alternatives for protecting plants against microbes, feeding herbivores, and weeds. Terpenes are the largest among PSMs and have been extensively studied for their potential as antimicrobial, insecticidal, and weed control agents. They also attract natural enemies of pests and beneficial insects, such as pollinators and dispersers. However, most of these research findings are shelved and fail to pass beyond the laboratory and greenhouse stages. This review provides an overview of terpenes, types, biosynthesis, and their roles in protecting plants against microbial pathogens, insect pests, and weeds to rekindle the debate on using terpenes for the development of environmentally friendly biopesticides and herbicides. Keywords: terpenes; biosynthesis; phytoalexin; insecticidal; allelopathy Citation: Ninkuu, V.; Zhang, L.; Yan, J.; Fu, Z.; Yang, T.; Zeng, H. Biochemistry of Terpenes and Recent 1. Introduction Advances in Plant Protection. Int. J. Plants and a multitude of pathogenic microbes are in a constant battle for supremacy. -
Transcriptomic Analysis Reveals Potential Genes Involved in Tanshinone Biosynthesis in Salvia Miltiorrhiza Yujie Chang1,2, Meizhen Wang1, Jiang Li3 & Shanfa Lu 1*
www.nature.com/scientificreports OPEN Transcriptomic analysis reveals potential genes involved in tanshinone biosynthesis in Salvia miltiorrhiza Yujie Chang1,2, Meizhen Wang1, Jiang Li3 & Shanfa Lu 1* Tanshinones are important bioactive components in Salvia miltiorrhiza and mainly accumulate in the periderms of mature roots. Tanshinone biosynthesis is a complicated process, and little is known about the third stage of the pathway. To investigate potential genes that are responsible for tanshinone biosynthesis, we conducted transcriptome profling analysis of two S. miltiorrhiza cultivars. Diferential expression analysis provided 2,149 diferentially expressed genes (DEGs) for further analysis. GO and KEGG analysis showed that the DEGs were mainly associated with the biosynthesis of secondary metabolites. Weighted gene coexpression network analysis (WGCNA) was further performed to identify a “cyan” module associated with tanshinone biosynthesis. In this module, 25 cytochromes P450 (CYPs), three 2-oxoglutarate-dependent dioxygenases (2OGDs), one short-chain alcohol dehydrogenases (SDRs) and eight transcription factors were found to be likely involved in tanshinone biosynthesis. Among these CYPs, 14 CYPs have been reported previously, and 11 CYPs were identifed in this study. Expression analysis showed that four newly identifed CYPs were upregulated upon application of MeJA, suggesting their possible roles in tanshinone biosynthesis. Overall, this study not only identifed candidate genes involved in tanshinone biosynthesis but also provided a basis for characterization of genes involved in important active ingredients of other traditional Chinese medicinal plants. Salvia miltiorrhiza (typically called danshen in Chinese) belongs to the Lamiaceae family and is a commonly used traditional Chinese medicine with great economic and medicinal value. Te S. miltiorrhiza root has been widely used for the treatment of cardiovascular and cerebrovascular diseases1. -
Monoterpene and Sesquiterpene Synthases and the Origin of Terpene Skeletal Diversity in Plants
Phytochemistry 70 (2009) 1621–1637 Contents lists available at ScienceDirect Phytochemistry journal homepage: www.elsevier.com/locate/phytochem Review Monoterpene and sesquiterpene synthases and the origin of terpene skeletal diversity in plants Jörg Degenhardt a,*, Tobias G. Köllner a, Jonathan Gershenzon b a Martin Luther University Halle-Wittenberg, Institute for Pharmacy, Hoher Weg 8, D-06120 Halle/Saale, Germany b Max Planck Institute for Chemical Ecology, Hans-Knöll Strasse 8, D-07745 Jena, Germany article info abstract Article history: The multitude of terpene carbon skeletons in plants is formed by enzymes known as terpene synthases. Received 10 March 2009 This review covers the monoterpene and sesquiterpene synthases presenting an up-to-date list of Received in revised form 23 July 2009 enzymes reported and evidence for their ability to form multiple products. The reaction mechanisms Available online 28 September 2009 of these enzyme classes are described, and information on how terpene synthase proteins mediate catal- ysis is summarized. Correlations between specific amino acid motifs and terpene synthase function are Keywords: described, including an analysis of the relationships between active site sequence and cyclization type Monoterpene synthase and a discussion of whether specific protein features might facilitate multiple product formation. Sesquiterpene synthase Ó 2009 Elsevier Ltd. All rights reserved. Terpene synthase reaction mechanism Site-directed mutagenesis Terpene synthase structure Structure–function correlation -
The Family of Terpene Synthases in Plants: a Mid-Size Family of Genes for Specialized Metabolism That Is Highly Diversified Throughout the Kingdom
The Plant Journal (2011) 66, 212–229 doi: 10.1111/j.1365-313X.2011.04520.x THE PLANT GENOME: AN EVOLUTIONARY VIEW ON STRUCTURE AND FUNCTION The family of terpene synthases in plants: a mid-size family of genes for specialized metabolism that is highly diversified throughout the kingdom Feng Chen1,*, Dorothea Tholl2,Jo¨ rg Bohlmann3 and Eran Pichersky4 1Department of Plant Sciences, University of Tennessee, Knoxville, TN 37996, USA, 2Department of Biological Sciences, 408 Latham Hall, Virginia Polytechnic Institute and State University, Blacksburg, VA 24061, USA, 3Michael Smith Laboratories, 2185 East Mall, University of British Columbia, Vancouver, BC V6T 1Z4, Canada, and 4Department of Molecular, Cellular and Developmental Biology, University of Michigan, Ann Arbor, MI 48109, USA Received 14 October 2010; revised 19 January 2011; accepted 31 January 2011. *For correspondence (fax +1 865 974 1947; e-mail [email protected]). SUMMARY Some plant terpenes such as sterols and carotenes are part of primary metabolism and found essentially in all plants. However, the majority of the terpenes found in plants are classified as ‘secondary’ compounds, those chemicals whose synthesis has evolved in plants as a result of selection for increased fitness via better adaptation to the local ecological niche of each species. Thousands of such terpenes have been found in the plant kingdom, but each species is capable of synthesizing only a small fraction of this total. In plants, a family of terpene synthases (TPSs) is responsible for the synthesis of the various terpene molecules from two isomeric 5-carbon precursor ‘building blocks’, leading to 5-carbon isoprene, 10-carbon monoterpenes, 15- carbon sesquiterpenes and 20-carbon diterpenes. -
Bacterial Diterpene Synthases: New Opportunities for Mechanistic
Available online at www.sciencedirect.com Bacterial diterpene synthases: new opportunities for mechanistic enzymology and engineered biosynthesis 1 2,3 3 Michael J Smanski , Ryan M Peterson , Sheng-Xiong Huang and 1,2,3,4,5 Ben Shen Diterpenoid biosynthesis has been extensively studied in plants potential for terpenoids in bacteria, particularly in the and fungi, yet cloning and engineering diterpenoid pathways in actinomycetes, may be significantly underestimated [1,2]. these organisms remain challenging. Bacteria are emerging as prolific producers of diterpenoid natural products, and bacterial Diterpenoid biosynthesis has been extensively studied diterpene synthases are poised to make significant in plants and fungi [3–6], yet cloning the respective contributions to our understanding of terpenoid biosynthesis. genes and characterizing and engineering diterpenoid Here we will first survey diterpenoid natural products of pathways in these higher organisms remain challenging bacterial origin and briefly review their biosynthesis with [7,8]. Scattering of the biosynthetic genes on the geno- emphasis on diterpene synthases (DTSs) that channel mic DNA of these higher organisms substantially geranylgeranyl diphosphate to various diterpenoid scaffolds. increases the effort to clone all the genes encoding We will then highlight differences of DTSs of bacterial and the complete biosynthetic machinery for a given diter- higher organism origins and discuss the challenges in penoid natural product. By contrast, genes encoding discovering novel bacterial DTSs. We will conclude by secondary metabolite biosynthesis in actinomycetes are discussing new opportunities for DTS mechanistic enzymology nearly always arranged on the bacterial chromosome as and applications of bacterial DTS in biocatalysis and metabolic a cluster. Recent characterization of terpene synthases pathway engineering. -
A Fungal Diterpene Synthase Is Responsible for Sterol Biosynthesis for Growth
fmicb-11-01426 July 8, 2020 Time: 19:11 # 1 ORIGINAL RESEARCH published: 10 July 2020 doi: 10.3389/fmicb.2020.01426 A Fungal Diterpene Synthase Is Responsible for Sterol Biosynthesis for Growth Yanjie Liu1, Anqing Duan1, Longfei Chen2,3, Dan Wang3, Qiaohong Xie1, Biyun Xiang1, Yamin Lin1, Xiaoran Hao1 and Xudong Zhu1* 1 Beijing Key Laboratory of Genetic Engineering Drug and Biotechnology, Institute of Biochemistry and Biotechnology, College of Life Sciences, Beijing Normal University, Beijing, China, 2 Zhejiang Medicine Co., Ltd., Zhejiang, China, 3 Department of Microbiology, College of Life Sciences, Nankai University, Tianjin, China A conserved open reading frame, dps, is described in Pestalotiopsis microspora, sharing a remarkable similarity with fungal diterpene synthases whose function is less studied. Loss-of-function approach manifested that dps was necessary for the growth and the development of the fungus. A deletion strain, dps1, showed a fundamental retardation in growth, which could deliberately be restored by the addition of exogenous sterols to the media. Gas chromatography–mass spectrometry analysis confirmed the loss of Edited by: the ability to produce certain sterols. Thus, the tolerance and the resistance of dps1 to Anindya Chanda, several stress conditions were impaired. Secondary metabolites, such as the polyketide University of South Carolina, derivative dibenzodioxocinones, were significantly diminished. At the molecular level, the United States deletion of dps even affected the expression of genes in the mevalonate pathway. This Reviewed by: Christoph Müller, report adds knowledge about fungal diterpene synthases in Pestalitiopsis microspora. Ludwig Maximilian University of Munich, Germany Keywords: Pestalotiopsis microspora, diterpene synthase, MVA genes, sterol biosynthesis, polyketide Ashutosh Singh, University of Lucknow, India INTRODUCTION *Correspondence: Xudong Zhu [email protected] Terpenoids are known as one of the most abundant natural products (Ruan et al., 2019). -
Chemistry of Secondary Metabolites
Review Article Annals of Clinical Toxicology Published: 31 May, 2019 Chemistry of Secondary Metabolites Mohiuddin AK* Department of Pharmacy, World University of Bangladesh, Bangladesh Abstract Medicinal plants, are known to deliver a wide scope of Plant Secondary Metabolites (PSMs) connected as bug sprays, medications, colors and poisons in farming, prescription, industry and bio- fighting in addition to bio-fear mongering, separately. Be that as it may, creation of PSMs is for the most part in little amounts, so we have to discover novel approaches to increment both amount and nature of them. Luckily, biotechnology proposes a few choices through which secondary metabolism in plants can be built in imaginative approaches to: 1) over-produce the valuable metabolites, 2) down-produce the poisonous metabolites, 3) produce the new metabolites. Secondary metabolites are extensively characterized as natural products integrated by a life form that is not basic to help development and life. The plant kingdom fabricates more than 200,000 unmistakable chemical compounds, a large portion of which emerge from particular metabolism. While these compounds assume critical jobs in interspecies challenge and safeguard, many plant natural products have been abused for use as prescriptions, scents, flavors, supplements, repellants, and colorants. In spite of this immense chemical decent variety, numerous secondary metabolites are available at low focuses in plant a, taking out yield based assembling as methods for achieving these imperative products. The basic and stereo chemical unpredictability of particular metabolites frustrates most endeavors to get to these compounds utilizing chemical blend. Albeit local plants can be built in amass target pathway metabolites. -
Terpenoid-Based Defenses in Conifers: Cdna Cloning
Proc. Natl. Acad. Sci. USA Vol. 95, pp. 6756–6761, June 1998 Biochemistry Terpenoid-based defenses in conifers: cDNA cloning, characterization, and functional expression of wound-inducible (E)-a-bisabolene synthase from grand fir (Abies grandis) [sesquiterpene synthaseyjuvenile hormone analogueyplant defense geney(E)-4-(1,5-dimethyl-1,4-hexadienyl)-1-methylcyclohexeneytodomatuic acid] JO¨RG BOHLMANN*†,JOHN CROCK†,REINHARD JETTER‡, AND RODNEY CROTEAU§ Institute of Biological Chemistry and Department of Biochemistry and Biophysics, Washington State University, Pullman, WA 99164-6340 Contributed by Rodney Croteau, April 8, 1998 ABSTRACT (E)-a-Bisabolene synthase is one of two the structural analysis of the corresponding catalysts and to the wound-inducible sesquiterpene synthases of grand fir (Abies molecular dissection of induced monoterpene and diterpene grandis), and the olefin product of this cyclization reaction is biosynthesis (14, 15). considered to be the precursor in Abies species of todomatuic Comparatively little is known about the kinetics and func- acid, juvabione, and related insect juvenile hormone mimics. tional significance of sesquiterpene biosynthesis in conifers. A cDNA encoding (E)-a-bisabolene synthase was isolated Sesquiterpenes of the juvabione family, based on the bisabo- from a wound-induced grand fir stem library by a PCR-based lane skeleton (Fig. 1), mimic insect juvenile hormones and, strategy and was functionally expressed in Escherichia coli and thus, can disrupt insect development and reproduction (20, shown