Does the LMC Possess a Dark Bulge? A
Total Page:16
File Type:pdf, Size:1020Kb
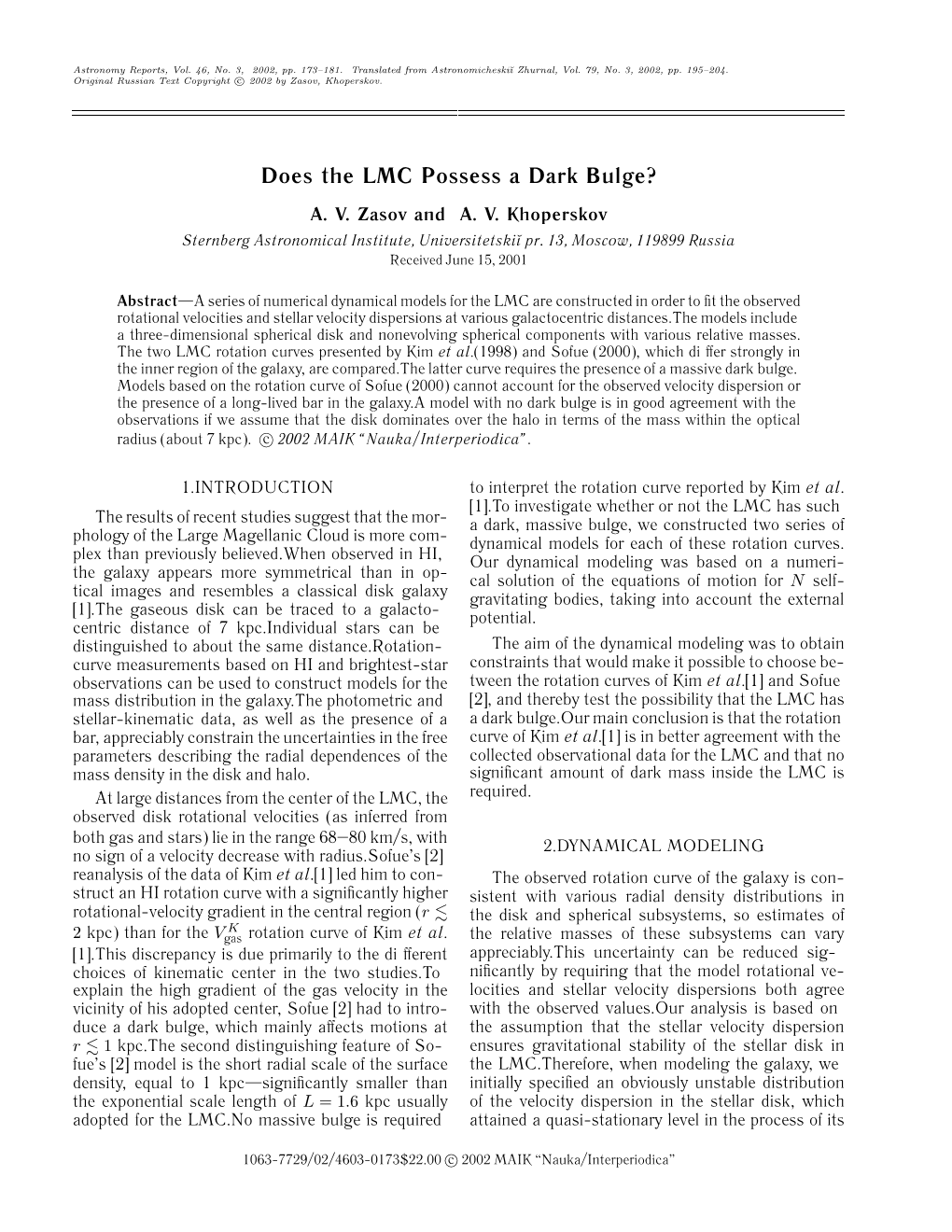
Load more
Recommended publications
-
Very High Energy Emission from Blazars Interpreted Through Simultaneous Multiwavelength Observations
UNIVERSITA` DEGLI STUDI DI SIENA FACOLTA` DI SCIENZE MATEMATICHE, FISICHE E NATURALI Dipartimento di Fisica Very High Energy emission from blazars interpreted through simultaneous multiwavelength observations Relatore/Supervisor: Candidato/Candidate: Dr. Antonio Stamerra Giacomo Bonnoli Tutore/Tutor: Prof. Riccardo Paoletti Ph.D. School in Physics Cycle XXI December 2010 Abstract In the framework of Astroparticle Physics the understanding of the particle acceleration process and related high energy electromagnetic emission within astrophysical sources is an issue of fundamental importance to unravel the structure and evolution of many classes of celestial objects, on different scales from micro{quasars to active galactic nuclei. This has an important role not only for astrophysics itself, but for many related topics of cosmic ray physics and High Energy physics, such as the search for dark matter. Also cosmology is interested, as deepening our knowledge on Active Galactic Nuclei and their interaction with the environment can help to clarify open issues on the formation of cosmic structures and evolution of universe on large scales. The present view on sources emitting high energy radiation is now gaining new insight thanks to multiwavelength observations. This approach allows to explore the spectral energy distribution of the sources all across the electromagnetic spectrum, therefore granting the best achievable understanding of the physical processes that originate the radiation that we see, and their mutual relationships. Our theories model the sources in terms of parameters that can be inferred from the observables quantities measured, and the multiwavelength observations are a key instrument in order to rule out or support some selected models out of the many that compete in the effort of describing the processes at work. -
Csillagászati Évkönyv 1970
CSILLAGÁSZATI ÉVKÖNYV 1970 CSILLAGÁSZATI ÉVKÖNYV A Z 1970. É V R E SZERKESZTETTE A TUDOMÁNYOS ISMERETTERJESZTŐ TÁRSULAT CSILLAGÁSZATI ÉS ŰRKUTATÁSI SZAKOSZTÁLYAINAK ORSZÁGOS VÁLASZTMÁNYA GONDOLAT KIADÓ n BUDAPEST 1969 /! J CSILLAGÁSZATI ADATOK AZ 1970. ÉVRE Az I—XVI. táblázatokat összeállította TIT Hajdú-Bihar Megyei Csillagászati Szakosztálya MTA Napfizikai Obszervatórium közreműködésével (Debrecen) T. JANUÁR KÖZÉP-EURÓPAI zónaidőben (KÖZÉI) Budapestéii ----------- A NAP A HOLD AHOLD fény változásai nyug nyug kel delel szik kel szik DÁTUM Év Év hányadiknapja A A HÉT napjai Év Év hányadik hete h m h m h m h m li m h m 1 Cs (1) i 7 32 11 47 16 03 0 05 11 17 2 P 2 7 32 11 48 16 04 1 17 11 34 3 Sz 3 7 32 11 49 16 05 2 34 11 55 4 V 4 7 32 11 49 16 06 3 55 12 24 5 H 2 5 7 32 11 50 16 07 5 18 13 05 6 K 6 7 32 11 50 16 09 6 34 14 02 7 Sz 7 7 31 11 51 16 10 7 38 15 18 <0 21 36 8 Cs 8 7 31 11 51 16 11 8 26 16 45 9 P 9 7 31 11 51 16 12 9 00 18 16 10 Sz 10 7 30 11 52 16 13 9 25 19 44 11 V 11 7 30 11 52 16 14 9 45 21 08 * 12 H 3 12 7 29 11 52 16 16 10 03 22 28 13 K 13 7 29 11 53 16 17 10 19 23 44 14 Sz 14 7 29 11 53 16 18 10 36 — ) 14 ] 8 15 Cs 15 7 28 11 54 16 20 10 55 1 01 16 P 16 7 27 11 54 16 21 11 17 2 16 17 Sz ,17 7 27 11 54 16 22 11 45 3 29 18 V 18 7 26 11 55 16 24 12 21 4 38 19 H 4 19 7 25 11 55 16 25 13 07 5 40 20 K 20 7 24 11 55 16 27 14 04 6 31 21 Sz 21 7 23 11 56 16 28 15 08 7 11 22 Cs 22 7 22 11 56 16 30 16 19 7 42 0 1 3 55 23 P 23 7 21 11 56 16 31 17 25 8 06 24 Sz 24 7 20 11 56 16 32 18 32 8 25 25 V 25 7 19 11 56 16 34 19 39 8 41 26 H 5 26 7 18 11 57 16 35 20 46 8 55 27 K 27 7 17 11 57 16 37 21 53 9 09 28 Sz 28 7 16 11 57 16 38 23 03 9 22 29 Cs 29 7 15 11 57 16 40 — 9 38 30 P 30 7 14 11 57 16 41 0 16 9 57 ( 15 39 31 Sz 31 7 13 11 58 16 43 1 33 10 21 Hold: 8-án 1 lh-ltor földközelben 22-én 21h-kor földtávolban 4 HÓNAP Oh világidőkor NAP HOLD Júlián Csillagidő dátum (A = 0»-nál) 2440. -
1945Apj. . .102. .318S SIX-COLOR PHOTOMETRY of STARS III. THE
.318S SIX-COLOR PHOTOMETRY OF STARS .102. III. THE COLORS OF 238 STARS OF DIFFERENT SPECTRAL TYPES* Joel Stebbins1 and A. E. Whiteord2 1945ApJ. Mount Wilson Observatory and Washburn Observatory Received June 8,1945 ABSTRACT Colors have been obtained for 238 stars of all spectral types from O to M by measuring intensities i six spectral regions from X 3530 to X 10,300 A (Tables 2 and 3). The early-type stars from O to B3 sho small dispersion in intrinsic color, but many are strongly affected by space reddening. A dozen late-tyx giants in low latitudes are likewise affected. The most marked effect of absolute magnitude is near spe< trum K0, where the colors of dwarfs, ordinary giants, and supergiants are all different {Fig. 1). The observed colors of the stars agree closely with the colors of a black body at suitable temperatur« (Fig. 2). The derived relative color temperatures are based upon the mean of ten stars of spectrum dG with an assumed temperature of 5500°K. On this scale the values are 23,000° for O stars, 11,000° for A( and 5950° for dGO. An alternative scale, with 6700° and spectrum dG2 for the sun, gives 140,000° fc O stars, 16,000° for A0, and 6900° for dGO (Table 7). A definitive zero point for the temperature seal has not been determined. The bluest O and B stars agree very well with each other, but there is still the possibility that all ai slightly affected by space reddening. A dozen bright stars of the Pleiades seem normal for their type. -
Planetary Nebulae and Their Central Stars in the Magellanic Clouds 37 Eva Villaver, Letizia Stanghellini, and Richard A
Astrophysics and Space Science Proceedings The Impact of HST on European Astronomy F. Duccio Macchetto Editor Space Telescope Science Institute (STScI), Baltimore, MD 21218, USA Editor Dr. F. Duccio Macchetto Space Telescope Science Institute (STScI) 3700 San Martin Dr. Baltimore, MD 21218 USA [email protected] ISSN 1570-6591 e-ISSN 1570-6605 ISBN 978-90-481-3399-4 e-ISBN 978-90-481-3400-7 DOI 10.1007/978-90-481-3400-7 Springer Dordrecht Heidelberg London New York Library of Congress Control Number: 2009942446 © Springer Science+Business Media B.V. 2010 No part of this work may be reproduced, stored in a retrieval system, or transmitted in any form or by any means, electronic, mechanical, photocopying, microfilming, recording or otherwise, without written permission from the Publisher, with the exception of any material supplied specifically for the purpose of being entered and executed on a computer system, for exclusive use by the purchaser of the work. Cover design: eStudio Calamar S.L. Printed on acid-free paper Springer is part of Springer Science+Business Media (www.springer.com) Foreword Remembrance of Things Past It scarcely seems credible that it was almost exactly thirty years ago that I first met Duccio Macchetto at the first meeting of the newly formed Science Working Group of what was then called the Space Telescope project. We were there in slightly dif- ferent roles, Duccio as the project scientist for the Faint Object Camera and I as an interdisciplinary scientist. Henk van de Hulst was also there as the official repre- sentative of ESO. -
The Asiago Database on Photometric Systems (ADPS)?
ASTRONOMY & ASTROPHYSICS DECEMBER II 2000, PAGE 361 SUPPLEMENT SERIES Astron. Astrophys. Suppl. Ser. 147, 361–628 (2000) The Asiago Database on Photometric Systems (ADPS)? I. Census parameters for 167 photometric systems D. Moro1,2 and U. Munari1,3 1 Osservatorio Astronomico di Padova, Sede di Asiago, I-36012 Asiago (VI), Italy 2 Osservatorio Astrofisico di Asiago, Dipartimento di Astronomia, Universit`a di Padova, I-36012 Asiago (VI), Italy 3 CISAS, Centro Interdipartimentale di Studi ed Attivit`a Spaziali, Padova, Italy Received May 9; accepted August 14, 2000 Abstract. The Asiago Database on Photometric Systems Bessell (1993), in the books by Golay (1974) and Strai˘zys (ADPS) is a compilation of basic information and refer- (1995) and on the world wide web by Mermilliod et al. ence data on 167 optical, ultraviolet and infrared photo- (1997). The latter focuses mainly on the data collected in metric systems. Thirty-four additional systems are briefly various photometric systems (with entries for more than described. In compiling this census we have relied on pub- 2105 stars). At the same time, the Mermilliod et al. www lished information only. In Paper II the photometric sys- version available at the time of submission of the ADPS tems will be inter-compared, calibrated and parameterised provides a census of photometric systems by listing and by means of synthetic photometry using uniform criteria describing 82 of them. Even if our compilation was inde- and the same set of input spectra and extinction laws. pendent, we undoubtedly benefited by checking against Mermilliod et al.’s work. Key words: photometry — astronomical data bases — In compiling the ADPS we have tried to be as com- surveys plete as possible for the optical region (from 0.3 to 1.0 µm, where GAIA is currently planned to collect data), but ef- fort has also been made to include ultraviolet as well as infrared photometric systems (even if at a lower degree 1. -
Intermediate-Brightness Spectrophotometric Standards. Standards Near +40 Declination
Astronomy Reports, Vol. 45, No. 12, 2001, pp. 1002–1011. Translated from Astronomicheski˘ıZhurnal, Vol. 78, No. 12, 2001, pp. 1135–1145. Original Russian Text Copyright c 2001 by Tereshchenko. Intermediate-Brightness Spectrophotometric Standards. Standards Near +40◦ Declination V. M. Tereshchenko Fesenkov Astrophysical Institute, Academy of Sciences of Kazakhstan, Kamenskoe Plato, Almaty, 480068 Kazakhstan Received June 22, 2000 Abstract—The second stage in our program to compile a list of regional intermediate-brightness spec- trophotometric standards has been completed. We have obtained spectral energy distributions for 24 stars with magnitudes 7m. 0–8m. 5 near +40◦ declination. The range λλ3100–7600 A˚ was studied with a spectral resolution of 50 A.˚ The relative rms error of our results in the visible is 1–2%, increasing to 3–5% toward the edges of the studied wavelength interval. All the stars are referenced to a single standard, the circumpo- lar star HD 221525. The energy distributions were used to compute color indices in the UBV, WBVR,and UPXYZVS systems, as well as in the system of the TYCHO catalog. The computed and observed values for stars in common with the TYCHO catalog are compared. c 2001 MAIK “Nauka/Interperiodica”. 1. INTRODUCTION papers on spectrophotometry of faint stars present A star’s spectral energy distribution E(λ)isoneof data on fluxes or illuminations in the visible only for its principal observed, as well as physical, parameters. some wavelengths. Usually, the number of measured In addition, stars with well-studied energy distribu- positions in the spectrum does not exceed 20. tions can be used as spectrophotometric standards In [11], we proposed several stellar spectropho- for observations of other celestial bodies.