Free Electron Density Distribution of the Milky Way
Total Page:16
File Type:pdf, Size:1020Kb
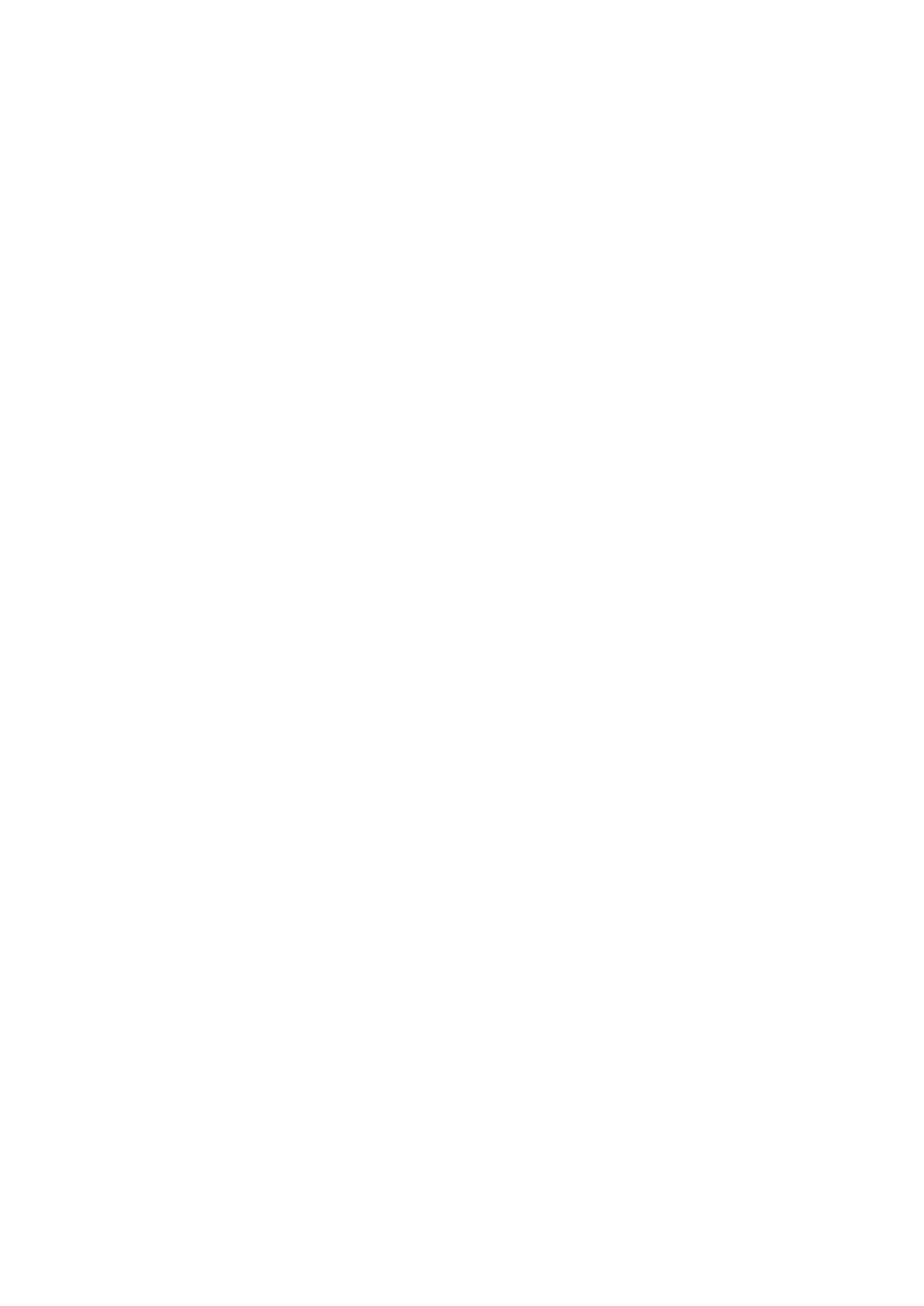
Load more
Recommended publications
-
High-Mass Starless Clumps in the Inner Galactic Plane: the Sample and Dust Properties Jinghua Yuan, Yuefang Wu, Simon P
High-mass Starless Clumps in the Inner Galactic Plane: The Sample and Dust Properties Jinghua Yuan, Yuefang Wu, Simon P. Ellingsen, Neal J. Evans, Christian Henkel, Ke Wang, Hong-li Liu, Tie Liu, Jin-zeng Li, Annie Zavagno To cite this version: Jinghua Yuan, Yuefang Wu, Simon P. Ellingsen, Neal J. Evans, Christian Henkel, et al.. High- mass Starless Clumps in the Inner Galactic Plane: The Sample and Dust Properties. Astrophysical Journal Supplement, American Astronomical Society, 2017, 231 (1), 10.3847/1538-4365/aa7204. hal- 01678391 HAL Id: hal-01678391 https://hal.archives-ouvertes.fr/hal-01678391 Submitted on 9 May 2018 HAL is a multi-disciplinary open access L’archive ouverte pluridisciplinaire HAL, est archive for the deposit and dissemination of sci- destinée au dépôt et à la diffusion de documents entific research documents, whether they are pub- scientifiques de niveau recherche, publiés ou non, lished or not. The documents may come from émanant des établissements d’enseignement et de teaching and research institutions in France or recherche français ou étrangers, des laboratoires abroad, or from public or private research centers. publics ou privés. HMSCs;Draft version March 2, 2018 Preprint typeset using LATEX style emulateapj v. 12/16/11 HIGH-MASS STARLESS CLUMPS IN THE INNER GALACTIC PLANE: THE SAMPLE AND DUST PROPERTIES Jinghua Yuan (袁lN)1y,Yuefang Wu (4月³)2,Simon P. Ellingsen3,Neal J. Evans II4,5,Christian Henkel6,7, Ke Wang (王科)8,Hong-Li Liu (刘*<)1,Tie Liu (刘Á)5,Jin-Zeng Li (N金增)1,Annie Zavagno9 1National Astronomical Observatories, -
A New Spiral Arm of the Galaxy: the Far 3-Kpc Arm T
APJLETTERS, ACCEPTED 7/9/08 Preprint typeset using LATEX style emulateapj v. 08/13/06 A NEW SPIRAL ARM OF THE GALAXY: THE FAR 3-KPC ARM T. M. DAME AND P. THADDEUS Harvard-Smithsonian Center for Astrophysics, 60 Garden Street, Cambridge MA 02138 [email protected], [email protected] ApJ Letters, accepted 7/9/08 ABSTRACT We report the detection in CO of the far-side counterpart of the well-known expanding 3-Kpc Arm in the central region of the Galaxy. In a CO longitude-velocity map at b = 0◦ the Far 3-Kpc Arm can be followed over at least 20◦ of Galactic longitude as a faint lane at positive velocities running parallel to the Near Arm. The Far Arm crosses l = 0◦ at +56 km s-1, quite symmetric with the -53 km s-1expansion velocity of the Near Arm. In addition to their symmetry in longitude and velocity, we find that the two arms have linewidths (∼ 21 km s-1), 6 -1 linear scale heights (∼ 103 pc FWHM), and H2 masses per unit length (∼ 4:3 x 10 M kpc ) that agree to 26% or better. Guided by the CO, we have also identified the Far Arm in high-resolution 21 cm data and find, subject to the poorly known CO-to-H2 ratio in these objects, that both arms are predominately molecular by a factor of 3–4. The detection of these symmetric expanding arms provides strong support for the existence of a bar at the center of our Galaxy and should allow better determination of the bar’s physical properties. -
The Observed Spiral Structure of the Milky Way⋆⋆⋆
A&A 569, A125 (2014) Astronomy DOI: 10.1051/0004-6361/201424039 & c ESO 2014 Astrophysics The observed spiral structure of the Milky Way, L. G. Hou and J. L. Han National Astronomical Observatories, Chinese Academy of Sciences, Jia-20, DaTun Road, ChaoYang District, 100012 Beijing, PR China e-mail: [lghou;hjl]@nao.cas.cn Received 21 April 2014 / Accepted 7 July 2014 ABSTRACT Context. The spiral structure of the Milky Way is not yet well determined. The keys to understanding this structure are to in- crease the number of reliable spiral tracers and to determine their distances as accurately as possible. HII regions, giant molecular clouds (GMCs), and 6.7 GHz methanol masers are closely related to high mass star formation, and hence they are excellent spiral tracers. The distances for many of them have been determined in the literature with trigonometric, photometric, and/or kinematic methods. Aims. We update the catalogs of Galactic HII regions, GMCs, and 6.7 GHz methanol masers, and then outline the spiral structure of the Milky Way. Methods. We collected data for more than 2500 known HII regions, 1300 GMCs, and 900 6.7 GHz methanol masers. If the photo- metric or trigonometric distance was not yet available, we determined the kinematic distance using a Galaxy rotation curve with the −1 −1 current IAU standard, R0 = 8.5 kpc and Θ0 = 220 km s , and the most recent updated values of R0 = 8.3 kpc and Θ0 = 239 km s , after velocities of tracers are modified with the adopted solar motions. With the weight factors based on the excitation parameters of HII regions or the masses of GMCs, we get the distributions of these spiral tracers. -
The SEDIGISM Survey: First Data Release and Overview of the Galactic Structure
ORE Open Research Exeter TITLE The SEDIGISM survey: First Data Release and overview of the Galactic structure AUTHORS Schuller, F; Urquhart, JS; Csengeri, T; et al. JOURNAL Monthly Notices of the Royal Astronomical Society DEPOSITED IN ORE 01 June 2021 This version available at http://hdl.handle.net/10871/125888 COPYRIGHT AND REUSE Open Research Exeter makes this work available in accordance with publisher policies. A NOTE ON VERSIONS The version presented here may differ from the published version. If citing, you are advised to consult the published version for pagination, volume/issue and date of publication MNRAS 500, 3064–3082 (2021) doi:10.1093/mnras/staa2369 Advance Access publication 2020 September 11 The SEDIGISM survey: First Data Release and overview of the Galactic structure F. Schuller ,1,2,3‹ J. S. Urquhart ,4‹ T. Csengeri,1,5 D. Colombo,1 A. Duarte-Cabral ,6 M. Mattern,1 A. Ginsburg,7 A. R. Pettitt ,8 F. Wyrowski,1 L. Anderson,9 F. Azagra,2 P. Barnes,10 M. Beltran,11 H. Beuther,12 S. Billington ,4 L. Bronfman,13 R. Cesaroni,11 C. Dobbs,14 D. Eden ,15 M.-Y. Lee,16 S.-N.X.Medina,1 K. M. Menten,1 T. Moore,15 F. M. Montenegro-Montes,2 S. Ragan ,6 A. Rigby ,6 M. Riener,12 D. Russeil,17 E. Schisano ,18 A. Sanchez-Monge,19 A. Traficante ,18 A. Zavagno,17 2 5 13 20 2 21 12 C. Agurto, S. Bontemps, R. Finger, A. Giannetti, E. Gonzalez, A. K. Hernandez, T. Henning, Downloaded from https://academic.oup.com/mnras/article/500/3/3064/5904091 by guest on 28 May 2021 J. -
Read Book 400 Billion Stars Kindle
400 BILLION STARS PDF, EPUB, EBOOK Paul McAuley | 240 pages | 14 Oct 2009 | Orion Publishing Co | 9780575090033 | English | London, United Kingdom 400 Billion Stars PDF Book So, all in all, a good read and McAuley has easily become one of my most favorite writers working today. Astronomical Society of the Pacific Conference Series. January 9, It's an impressive debut for Paul McCauley, and almost more along the lines of a novella rather than a space opera. At this speed, it takes around 1, years for the Solar System to travel a distance of 1 light-year, or 8 days to travel 1 AU astronomical unit. Archived from the original on September 16, Dame; P. Milky Way. Retrieved September 15, Bibcode : NatAs. Archived PDF from the original on May 14, Here, an elongated object such as a galaxy is viewed through an elongated slit, and the light is refracted using a device such as a prism. Main article: Local Group. Archived from the original on November 5, In , a star in the galactic halo, HE , was estimated to be about Return to Book Page. February 13, Carina-Sagittarius Arm. The Huffington Post. If you picked the quarter as being the average mass of a single coin, you might get one answer for the total number of coins. She's also a scientist, and when a small planet begins to manifest some unusual signs she is sent to investigate. If these arms contain an overdensity of stars compared to the average density of stars in the Galactic disk, it would be detectable by counting the stars near the tangent point. -
Photometric Distances to Young Stars in the Inner Galactic Disk II
A&A 548, A125 (2012) Astronomy DOI: 10.1051/0004-6361/201219653 & c ESO 2012 Astrophysics Photometric distances to young stars in the inner Galactic disk II. The region towards the open cluster Trumpler 27 at L = 355◦,, G. Perren1,R.A.Vázquez2, and G. Carraro3,4 1 Instituto de Física de Rosario, IFIR (CONICET-UNR), Parque Urquiza, 2000 Rosario, Argentina e-mail: [email protected] 2 Facultad de Ciencias Astronómicas y Geofísicas (UNLP), Instituto de Astrofísica de La Plata (CONICET, UNLP), Paseo del Bosque s/n, La Plata, Argentina e-mail: [email protected] 3 ESO, Alonso de Cordova 3107, Casilla 19100 Santiago de Chile, Chile e-mail: [email protected] 4 Dipartimento di Astronomia, Universita’ di Padova, Vicolo Osservatorio 5, 35122 Padova, Italy Received 23 May 2012 / Accepted 17 September 2012 ABSTRACT Context. The spiral structure of the Milky Way inside the solar circle is still poorly known because of the high density of the material that causes strong extinction towards the Galactic center. Aims. We present results of the first extensive and deep color–color diagram (CCD) photometric survey carried out in the field of the open cluster Trumpler 27, an object immersed in a region of extremely high visual absorption in the constellation of Sagittarius not far from the Galaxy center. The survey covers almost a quarter of square degree. Methods. We look for young stars clumps that might plausibly be associated with spiral structure. Wide-field UBVI photometry combined with infrared information allows us to reconstruct the distribution in the reddening and distance of young stars in the field using the CCD and color–magnitude diagrams (CMD). -
The Environmental Impact of High- and Low-Mass Stars: from Formation to Main Sequence
c 2013 by Ian William Stephens. All rights reserved. THE ENVIRONMENTAL IMPACT OF HIGH- AND LOW-MASS STARS: FROM FORMATION TO MAIN SEQUENCE BY IAN WILLIAM STEPHENS DISSERTATION Submitted in partial fulfillment of the requirements for the degree of Doctor of Philosophy in Astronomy in the Graduate College of the University of Illinois at Urbana-Champaign, 2013 Urbana, Illinois Doctoral Committee: Associate Professor Leslie W. Looney, Chair Professor You-Hua Chu Professor Emeritus Richard M. Crutcher Associate Professor Tony Wong Abstract Throughout the entire lifetime of a star, it continuously alters the environment. Diverse processes are involved which complicates studies of stellar interaction. This thesis focuses on two different physical phenomena associated with stars: (1) the interplay of magnetic fields and collapsing clouds, and (2) the effects of radiation from massive stars. We first compare polarization measurements of 52 Galactic star-forming regions with their locations in the Galaxy. In particular, we find that there is no correlation between the average magnetic field direction of star-forming molecular clouds and the Galaxy, indicating that star formation may eventually become its own process independent of the Galaxy. Secondly, we observe the coupling of the magnetic field with the low-mass protostar L1157-mm by creating polarimetric maps at resolutions from 300 to 2500 AU. The inferred magnetic field lines show a well-defined hourglass morphology centered ∼ about the core – only the second of such morphology discovered around a low-mass protostar. Next, we focus on radiation from massive stars in the Large Magellanic Cloud. We first present a survey of H II regions around massive young stellar objects (YSOs) and explore numerous relationship between parameters measured through observations of free-free and infrared emission. -
254 — 13 February 2014 Editor: Bo Reipurth ([email protected]) List of Contents
THE STAR FORMATION NEWSLETTER An electronic publication dedicated to early stellar/planetary evolution and molecular clouds No. 254 — 13 February 2014 Editor: Bo Reipurth ([email protected]) List of Contents The Star Formation Newsletter Interview ...................................... 3 My Favorite Object ............................ 5 Editor: Bo Reipurth [email protected] Perspective ................................... 10 Technical Editor: Eli Bressert Abstracts of Newly Accepted Papers .......... 13 [email protected] Abstracts of Newly Accepted Major Reviews . 54 Technical Assistant: Hsi-Wei Yen Dissertation Abstracts ........................ 60 [email protected] New Jobs ..................................... 61 Editorial Board Meetings ..................................... 63 Summary of Upcoming Meetings ............. 66 Joao Alves Alan Boss Short Announcements ........................ 67 Jerome Bouvier New Books ................................... 68 Lee Hartmann Thomas Henning Paul Ho Jes Jorgensen Charles J. Lada Cover Picture Thijs Kouwenhoven Michael R. Meyer This image shows the blueshifted outflow cav- Ralph Pudritz ity from the embedded quadruple Class I source Luis Felipe Rodr´ıguez L1551 IRS5 based on Hα and [SII] images obtained Ewine van Dishoeck with the Subaru telescope images. Two short jets, Hans Zinnecker HH 154, are seen to emerge from the source region to the upper left. The outflow cavity has burst The Star Formation Newsletter is a vehicle for through the front of the cloud, exposing the rich fast distribution of information of interest for as- and complex Herbig-Haro shock structures within. tronomers working on star and planet formation A few faint knots from the HH 30 jet (outside the and molecular clouds. You can submit material field) are visible at the top of the image. The for the following sections: Abstracts of recently field is about 7×8 arcmin, corresponding to about accepted papers (only for papers sent to refereed 0.30×0.35 pc at the assumed distance of 150 pc. -
Search for Age Pattern Across Spiral Arms of the Milky Way
RAA 2021 Vol. 21 No. 1, 9(10pp) doi: 10.1088/1674-4527/21/1/9 R c 2021 National Astronomical Observatories, CAS and IOP Publishing Ltd. esearch in Astronomy and http://www.raa-journal.org http://iopscience.iop.org/raa Astrophysics Search for age pattern across spiral arms of the Milky Way 1,2 1 3,4 £÷ Åð ûáf Zhi-Hong He (Û ) , Ye Xu ( ) and Li-Gang Hou ( ) 1 Purple Mountain Observatory, Chinese Academy of Sciences, Nanjing 210023, China 2 University of Science and Technology of China, Hefei 230026, China 3 National Astronomical Observatories, Chinese Academy of Sciences, Beijing 100101, China 4 CAS Key Laboratory of FAST, National Astronomical Observatories, Chinese Academy of Sciences, Beijing 100101, China Received 2020 July 16; accepted 2020 September 1 Abstract The age pattern across spiral arms is one of the key observational features utilised to study the dynamic nature of the Galaxy’s spiral structure. With the most updated samples of high-mass star formation region (HMSFR) masers, O stars and open clusters, we investigated their distributions and kinematic properties in the vicinity of the Sun. We found that the Sagittarius-Carina Arm traced by HMSFRs, O stars (. 10 Myr) and young open clusters (<30 Myr) seem to deviate gradually towards the Galactic Anticenter (GAC) direction. The Local Arm traced by HMSFRs, O stars, young clusters and also medium- young clusters (30−100 Myr) are inclined to gradually deviate toward the Galactic Center (GC) direction. The properties for the Local Arm are supported by a simplified simulation of cluster motions in the Galaxy. -
CHIMPS2: Survey Description and ¹²CO Emission in the Galactic Centre
This is a repository copy of CHIMPS2: survey description and ¹²CO emission in the Galactic Centre. White Rose Research Online URL for this paper: http://eprints.whiterose.ac.uk/171436/ Version: Accepted Version Article: Eden, DJ, Moore, TJT, Currie, MJ et al. (103 more authors) (2020) CHIMPS2: survey description and ¹²CO emission in the Galactic Centre. Monthly Notices of the Royal Astronomical Society, 498 (4). pp. 5936-5951. ISSN 0035-8711 https://doi.org/10.1093/mnras/staa2734 © 2020 The Author(s). Published by Oxford University Press on behalf of the Royal Astronomical Society. This is an author produced version of a paper published in Monthly Notices of the Royal Astronomical Society. Uploaded in accordance with the publisher's self-archiving policy. Reuse Items deposited in White Rose Research Online are protected by copyright, with all rights reserved unless indicated otherwise. They may be downloaded and/or printed for private study, or other acts as permitted by national copyright laws. The publisher or other rights holders may allow further reproduction and re-use of the full text version. This is indicated by the licence information on the White Rose Research Online record for the item. Takedown If you consider content in White Rose Research Online to be in breach of UK law, please notify us by emailing [email protected] including the URL of the record and the reason for the withdrawal request. [email protected] https://eprints.whiterose.ac.uk/ MNRAS 000, 1–18 (2020) Preprint 14 September 2020 Compiled using MNRAS LATEX style file v3.0 CHIMPS2: Survey description and 12CO emission in the Galactic Centre D. -
A New Spiral Arm of the Galaxy: the Far 3-Kpc Arm T
APJLETTERS, ACCEPTED 7/9/08 Preprint typeset using LATEX style emulateapj v. 08/22/09 A NEW SPIRAL ARM OF THE GALAXY: THE FAR 3-KPC ARM T. M. DAME AND P. THADDEUS Harvard-Smithsonian Center for Astrophysics, 60 Garden Street, Cambridge MA 02138 [email protected], [email protected] ApJ Letters, accepted 7/9/08 ABSTRACT We report the detection in CO of the far-side counterpart of the well-known expanding 3-Kpc Arm in the central region of the Galaxy. In a CO longitude-velocity map at b = 0◦ the Far 3-Kpc Arm can be followed over at least 20◦ of Galactic longitude as a faint lane at positive velocities running parallel to the Near Arm. The Far Arm crosses l = 0◦ at +56 km s-1, quite symmetric with the -53 km s-1expansion velocity of the Near Arm. In addition to their symmetry in longitude and velocity, we find that the two arms have linewidths (∼ 21 km s-1), 6 -1 linear scale heights (∼ 103 pc FWHM), and H2 masses per unit length (∼ 4:3 x 10 M kpc ) that agree to 26% or better. Guided by the CO, we have also identified the Far Arm in high-resolution 21 cm data and find, subject to the poorly known CO-to-H2 ratio in these objects, that both arms are predominately molecular by a factor of 3–4. The detection of these symmetric expanding arms provides strong support for the existence of a bar at the center of our Galaxy and should allow better determination of the bar’s physical properties. -
Evolution of Molecular and Atomic Gas Phases in The
The Astrophysical Journal, 823:76 (17pp), 2016 June 1 doi:10.3847/0004-637X/823/2/76 © 2016. The American Astronomical Society. All rights reserved. EVOLUTION OF MOLECULAR AND ATOMIC GAS PHASES IN THE MILKY WAY Jin Koda1,2, Nick Scoville2, and Mark Heyer3 1 Department of Physics and Astronomy, Stony Brook University, Stony Brook, NY 11794-3800, USA; [email protected] 2 California Institute of Technology, MC 249-17, 1200 East California Boulevard, Pasadena, CA 91125, USA 3 Department of Astronomy, University of Massachusetts, Amherst, MA 01003, USA Received 2015 October 9; accepted 2016 March 18; published 2016 May 24 ABSTRACT We analyze radial and azimuthal variations of the phase balance between the molecular and atomic interstellar medium (ISM) in the Milky Way (MW) using archival CO( J=1-0) and HI 21 cm data. In particular, the azimuthal variations—between the spiral arm and interarm regions—are analyzed without any explicit definition of the spiral arm locations. We show that the molecular gas mass fraction, i.e., fmol =SHHIH22() S +S , varies predominantly in the radial direction: starting from ~100% at the center, remaining 50% to R ~ 6 kpc and decreasing to ∼10%–20% at R = 8.5 kpc when averaged over the whole disk thickness (from ∼100% to ≳60%, then to ∼50% in the midplane). Azimuthal, arm-interarm variations are secondary: only ~20% in the globally molecule-dominated inner MW, but becoming larger, ∼40%–50%, in the atom-dominated outskirts. This suggests ( ) that in the inner MW the gas remains highly molecular fmol > 50% as it moves from an interarm region into a spiral arm and back into the next interarm region.