Geochronological Constraints on the Timing of Deformation: an Examination of the Prospect Rock Fault Footwall in North-Central V
Total Page:16
File Type:pdf, Size:1020Kb
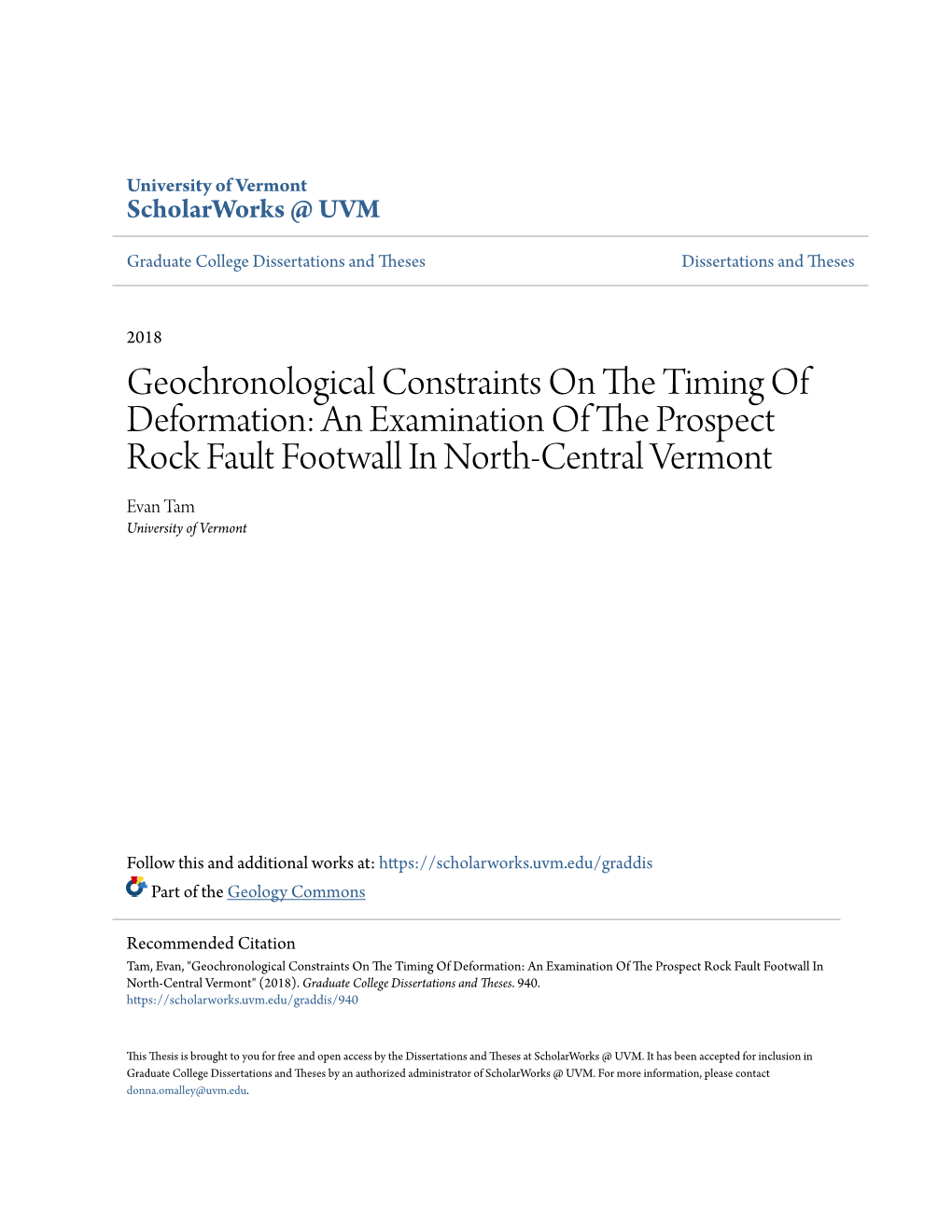
Load more
Recommended publications
-
Deformation Mechanisms, Rheology and Tectonics Geological Society Special Publications Series Editor J
Deformation Mechanisms, Rheology and Tectonics Geological Society Special Publications Series Editor J. BROOKS J/iLl THIS VOLUME IS DEDICATED TO THE WORK OF HENDRIK JAN ZWART GEOLOGICAL SOCIETY SPECIAL PUBLICATION NO. 54 Deformation Mechanisms, Rheology and Tectonics EDITED BY R. J. KNIPE Department of Earth Sciences Leeds University UK & E. H. RUTTER Department of Geology Manchester University UK ASSISTED BY S. M. AGAR R. D. LAW Department of Earth Sciences Department of Geological Sciences Leeds University Virginia University UK USA D. J. PRIOR R. L. M. VISSERS Department of Earth Sciences Institute of Earth Sciences Liverpool University University of Utrceht UK Netherlands 1990 Published by The Geological Society London THE GEOLOGICAL SOCIETY The Geological Society of London was founded in 1807 for the purposes of 'investigating the mineral structures of the earth'. It received its Royal Charter in 1825. The Society promotes all aspects of geological science by means of meetings, speeiat lectures and courses, discussions, specialist groups, publications and library services. It is expected that candidates for Fellowship will be graduates in geology or another earth science, or have equivalent qualifications or experience. Alt Fellows are entitled to receive for their subscription one of the Society's three journals: The Quarterly Journal of Engineering Geology, the Journal of the Geological Society or Marine and Petroleum Geology. On payment of an additional sum on the annual subscription, members may obtain copies of another journal. Membership of the specialist groups is open to all Fellows without additional charge. Enquiries concerning Fellowship of the Society and membership of the specialist groups should be directed to the Executive Secretary, The Geological Society, Burlington House, Piccadilly, London W1V 0JU. -
Faults and Joints
133 JOINTS Joints (also termed extensional fractures) are planes of separation on which no or undetectable shear displacement has taken place. The two walls of the resulting tiny opening typically remain in tight (matching) contact. Joints may result from regional tectonics (i.e. the compressive stresses in front of a mountain belt), folding (due to curvature of bedding), faulting, or internal stress release during uplift or cooling. They often form under high fluid pressure (i.e. low effective stress), perpendicular to the smallest principal stress. The aperture of a joint is the space between its two walls measured perpendicularly to the mean plane. Apertures can be open (resulting in permeability enhancement) or occluded by mineral cement (resulting in permeability reduction). A joint with a large aperture (> few mm) is a fissure. The mechanical layer thickness of the deforming rock controls joint growth. If present in sufficient number, open joints may provide adequate porosity and permeability such that an otherwise impermeable rock may become a productive fractured reservoir. In quarrying, the largest block size depends on joint frequency; abundant fractures are desirable for quarrying crushed rock and gravel. Joint sets and systems Joints are ubiquitous features of rock exposures and often form families of straight to curviplanar fractures typically perpendicular to the layer boundaries in sedimentary rocks. A set is a group of joints with similar orientation and morphology. Several sets usually occur at the same place with no apparent interaction, giving exposures a blocky or fragmented appearance. Two or more sets of joints present together in an exposure compose a joint system. -
The Geochemistry and Petrogenesis of the Paleoproterozoic Du Chef Dyke Swarm, Québec, Canada
This is an Open Access document downloaded from ORCA, Cardiff University's institutional repository: http://orca.cf.ac.uk/60323/ This is the author’s version of a work that was submitted to / accepted for publication. Citation for final published version: Ciborowski, Thomas, Kerr, Andrew Craig, McDonald, Iain, Ernst, Richard E., Hughes, Hannah S. R. and Minifie, Matthew John 2014. The geochemistry and petrogenesis of the Paleoproterozoic du Chef dyke swarm, Québec, Canada. Precambrian Research 250 , pp. 151-166. 10.1016/j.precamres.2014.05.008 file Publishers page: http://dx.doi.org/10.1016/j.precamres.2014.05.008 <http://dx.doi.org/10.1016/j.precamres.2014.05.008> Please note: Changes made as a result of publishing processes such as copy-editing, formatting and page numbers may not be reflected in this version. For the definitive version of this publication, please refer to the published source. You are advised to consult the publisher’s version if you wish to cite this paper. This version is being made available in accordance with publisher policies. See http://orca.cf.ac.uk/policies.html for usage policies. Copyright and moral rights for publications made available in ORCA are retained by the copyright holders. The geochemistry and petrogenesis of the Paleoproterozoic du Chef dyke swarm, Québec, Canada T. Jake. R. Ciborowski*1, Andrew C. Kerr1, Iain McDonald1, Richard E. Ernst2, Hannah S. R. Hughes1, and Matthew J. Minifie1. 1School of Earth and Ocean Sciences, Cardiff University, Park Place, Cardiff, Wales, CF10 3AT, UK 2Department of Earth Sciences, Carleton University, 1125 Colonel By Drive, Ottawa, Ontario, K1S 5B6, Canada & Ernst Geosciences, 43 Margrave Ave. -
Geology: Ordovician Paleogeography and the Evolution of the Iapetus Ocean
Ordovician paleogeography and the evolution of the Iapetus ocean Conall Mac Niocaill* Department of Geological Sciences, University of Michigan, 2534 C. C. Little Building, Ben A. van der Pluijm Ann Arbor, Michigan 48109-1063. Rob Van der Voo ABSTRACT thermore, we contend that the combined paleomagnetic and faunal data ar- Paleomagnetic data from northern Appalachian terranes identify gue against a shared Taconic history between North and South America. several arcs within the Iapetus ocean in the Early to Middle Ordovi- cian, including a peri-Laurentian arc at ~10°–20°S, a peri-Avalonian PALEOMAGNETIC DATA FROM IAPETAN TERRANES arc at ~50°–60°S, and an intra-oceanic arc (called the Exploits arc) at Displaced terranes occur along the extent of the Appalachian-Cale- ~30°S. The peri-Avalonian and Exploits arcs are characterized by Are- donian orogen, although reliable Ordovician paleomagnetic data from Ia- nigian to Llanvirnian Celtic fauna that are distinct from similarly aged petan terranes have only been obtained from the Central Mobile belt of the Toquima–Table Head fauna of the Laurentian margin, and peri- northern Appalachians (Table 1). The Central Mobile belt separates the Lau- Laurentian arc. The Precordillera terrane of Argentina is also charac- rentian and Avalonian margins of Iapetus and preserves remnants of the terized by an increasing proportion of Celtic fauna from Arenig to ocean, including arcs, ocean islands, and ophiolite slivers (e.g., Keppie, Llanvirn time, which implies (1) that it was in reproductive communi- 1989). Paleomagnetic results from Arenigian and Llanvirnian volcanic units cation with the peri-Avalonian and Exploits arcs, and (2) that it must of the Moreton’s Harbour Group and the Lawrence Head Formation in cen- have been separate from Laurentia and the peri-Laurentian arc well tral Newfoundland indicate paleolatitudes of 11°S (Table 1), placing them before it collided with Gondwana. -
The Taconic Orogeny in Newfoundland: a Three-Stage Process
atlantic geology . volume 43 . 2007 83 geochronology and isotope geology, indicated that this model was incomplete. We will present new evidence that the Taconic orogeny comprises three separate accretionary events starting in the Late Cambrian and finishing in the Late Ordovician. Taconic 1 is represented by ca. 495 Ma west-directed obduc- tion of the ca. 510 Ma Lushs Bight oceanic Tract onto the peri- Laurentian Dashwoods microcontinent. Subduction is inferred to have initiated at a spreading centre abandoned during an inboard ridge jump responsible for separation of Dashwoods from Laurentia. Partial subduction of the buoyant Dashwoods forced subduction to step back into the Humber seaway, which led to formation of the ca. 490 Ma Baie Verte oceanic tract (BVOT). Dextral oblique closure of the Humber seaway first formed the Notre Dame arc (489–477 Ma) built on Dashwoods and the coeval Snooks Arm arc built on the BVOT, followed by their collision with Laurentia (Taconic 2) and each other. The obliquity of convergence induced large-scale translations of continental ribbons of the Laurentian margin from the lati- tude of Labrador to central Newfoundland. After a magmatic gap of c. 7–10 my, the Notre Dame arc records a voluminous flare-up of predominantly tonalite magmatism (464–459 Ma) during the waning stages of Taconic 2. Magmatism overlaps with strong deformation and comprises both arc and non-arc- like tonalite. We relate this flare-up to break-off of the oceanic lithosphere of the downgoing Laurentian slab. Taconic 3 is rep- resented by 455–450 Ma collision between a peri-Laurentian arc terrane and the peri-Gondwanan Popelogan-Exploits arc and their composite accretion to Laurentia. -
Deformation at the Microscale
Deformation at the Microscale Deformation processes occur at the microscale and lead to changes in the internal structure, shape or volume of a rock. Brittle versus Plastic deformation mechanisms is a function of: • Pressure – increasing pressure favors plastic deformation • Temperature – increasing temperature favors plastic deformation • Rheology of the deforming minerals (for example quartz vs feldspar) • Availability of fluids – favors brittle deformation • Strain rate – lower strain rate favors plastic deformation Given the different rheology of different minerals, brittle and plastic deformation mechanisms can occur in the same sample under the same conditions. The controlling deformation mechanism determines whether the deformation belongs to the brittle or plastic regime. Brittle deformation mechanisms operative at shallow depths. Crystal-plastic Deformation Mechanisms Mechanical twining – mechanical bending or kinking of the crystal structure. Mechanical twins in calcite Deformation (glide) twins in a calcite crystal. Stress is ideally at 45o to the shear In the case of calcite, the 38o angle (glide plane). Dark lamellae turns the twin plane into a mirror have been sheared (simple plane. The amount of strain shear). associated with a single kink is fixed by this angle. Crystal Defects • Point defects – due to vacancies or impurities in the crystal structure. • Line defects (dislocations) – a mobile line defect that causes intracrystalline deformation via slip. The slip plane is usually the place in a crystal that has the highest density of atoms. • Plane defects – grain boundaries, subgrain boundaries, and twin planes. Migration of vacancies Types of defects – vacancy, through a crystal structure by substitution, interstitial diffusion. The movement of dislocations occurs in the plane or direction which requires the least energy. -
Paleozoic 3: Alabama in the Paleozoic
UNIVERSITY OF SOUTH ALABAMA GY 112: Earth History Paleozoic 3: Alabama in the Paleozoic Instructor: Dr. Douglas W. Haywick Last Time The Paleozoic Part 2 1) Back to Newfoundland 2) Eastern Laurentian Orogenies (Appalachians) 3) Other Laurentian Orogenies (Antler, Ouachita) (web notes 25) Laurentia (Paleozoic North America) Even though this coastline of Laurentia was a passive continental margin, a plate tectonic boundary was rapidly approaching… A B A B Laurentia (Paleozoic North America) The resulting Taconic Orogeny first depressed the seafloor Laurentia (localized transgression) and A Island arc then pushed previously deposited passive continental B margin sediments up into thrust fault mountains. Baltica There was only minimal metamorphism and igneous A intrusions. B Middle Ordovician Laurentia (Paleozoic North America) Laurentia Baltica Middle Ordovician Laurentia (Paleozoic North America) Laurentia Baltica Middle Ordovician Laurentia (Paleozoic North America) The next tectonic event (the Acadian Orogeny) was caused Laurentia by the approach of Baltica A B Baltica A B Baltica Baltica Late Ordovician Laurentia (Paleozoic North America) The Acadian Orogeny was more extensive and more intense (metamorphism and A lots of igneous intrusions) B A B Early Devonian Laurentia (Paleozoic North America) The Acadian Orogeny was more extensive and more intense (metamorphism and lots of igneous intrusions) Early Devonian Laurentia (Paleozoic North America) Lastly, along comes Gondwanna and…. …well you get the idea. A B B A B Mississippian Laurentia (Paleozoic North America) Lastly, along comes Gondwanna and…. …well you get the idea. A B B A B Pennsylvannian Suture zone Laurentia (Paleozoic North America) Lastly, along comes Gondwanna and…. …well you get the idea. -
70-26,250 University Microfilms, a XEROX Company, Ann Arbor
70-26,250 BICKEL, Edwin David, 1941- PLEISTOCENE NON-MARINE MOLLUSCA OF THE GATINEAU VALLEY AND OTTAWA AREAS OF QUEBEC AND ONTARIO, CANADA. The Ohio State University, Ph.D., 1970 Geology University Microfilms, A XEROX Company, Ann Arbor, Michigan PLEISTOCENE NON-KARINE NCLLUSCA OF THE GATINEAU VALLEY AND OTTAWA AREAS OF QUEBEC AND ONTARIO, CANADA DISSERTATION Presented in Partial Fulfillment of the Requirements for the Degree of Doctor of Philosophy In the Graduate School of The Ohio State University By Edwin David Bickel, A.B., M.Sc* * * * * * The Ohio State University 1970 Approved by r I' N J U u Adviser // Department of Geology ACKNOWLEDGMENTS I am most appreciative of the valuable guidance and assistance Professor Aurele La Rocque has given to this work and my other academic endeavors at The Ohio State University. Ky wife, Barbara Sue, deserves special recognition for many hours of skillful help in the field and laboratory. I wish to thank John G. Fyles and R, J. Kott of the Geological Survey of Canada, who generously made available samples and data gathered in conjunction with Dr. Mott*s research. Figure 3 from Geological Survey Memoir 2 M is reproduced as Fig. 2 of this paper by permission of the Geological Survey of Canada, Financial assistance for field work was provided by National Science Foundation Grant GB-818. I am grateful to the Friends of Orton Hall Fund for helping defray the cost of reproducing the illustrations. Finally, I wish to dedicate this dissertation to four teachers who have inspired and directed my scientific training. Professors Daniel F. -
Post-Seismic Deformation Mechanism of the July 2015 MW 6.5 Pishan
www.nature.com/scientificreports OPEN Post‑seismic deformation mechanism of the July 2015 MW 6.5 Pishan earthquake revealed by Sentinel‑1A InSAR observation Sijia Wang1*, Yongzhi Zhang1,2*, Yipeng Wang1, Jiashuang Jiao 1, Zongtong Ji1 & Ming Han1 On 3 July 2015, the Mw 6.5 Pishan earthquake occurred at the junction of the southwestern margin of the Tarim Basin and the northwestern margin of the Tibetan Plateau. To understand the seismogenic mechanism and the post‑seismic deformation behavior, we investigated the characteristics of the post‑seismic deformation felds in the seismic area, using 9 Sentinel‑1A TOPS synthetic aperture radar (SAR) images acquired from 18 July 2015 to 22 September 2016 with the Small Baseline Subset Interferometric SAR (SBAS‑InSAR) technique. Postseismic LOS deformation displayed logarithmic behavior, and the temporal evolution of the post‑seismic deformation is consistent with the aftershock sequence. The main driving mechanism of near‑feld post‑seismic displacement was most likely to be afterslip on the fault and the entire creep process consists of three creeping stages. Afterward, we used the steepest descent method to invert the afterslip evolution process and analyzed the relationship between post‑seismic afterslip and co‑seismic slip. The results witness that 447 days after the mainshock (22 September 2016), the afterslip was concentrated within one principal slip center. It was located 5–25 km along the fault strike, 0–10 km along with the fault dip, with a cumulative peak slip of 0.18 m. The 447 days afterslip seismic moment was approximately 2.65 × 1017 N m, accounting for approximately 4.1% of the co‑seismic geodetic moment. -
Geology and Topography of Dutchess County (.Pdf)
Chapter 3: The Geology and Topography of Dutchess County Chapter 3: Geology and Topography of Dutchess County, NY ______________________________________________________________________________ Roy T. Budnik, Jeffery R. Walker, and Kirsten Menking1 May 2010 INTRODUCTION The topography, settlement patterns, and mineral resources of Chapter Contents Dutchess County are all influenced by the underlying geology. Geologic History For example, the highest mountains contain the hardest rocks, Bedrock Formations Structural Geology communities in the county are generally located in areas of Surficial Deposits sand and gravel because of the relatively level terrain and Mineral Resources Topography abundant water supplies they contain, and construction Trends and Changes Over aggregates are mined where suitable deposits are found. Time Implications for Decision- Understanding geologic materials and processes is essential to Making sound resource management because the geology affects the Resources 1 This chapter was written during 2010 by Dr. Roy T. Budnik (President, Roy T. Budnik & Associates), Dr. Jeffrey R. Walker (Professor of Earth Science & Geography, Vassar College), and Dr. Kirsten Menking (Associate Professor of Earth Science and Geography, Vassar College). It is an updated and expanded version of the Hydrology chapter of the 1985 document Natural Resources, Dutchess County, NY (NRI). Natural Resource Inventory of Dutchess County, NY 1 Chapter 3: The Geology and Topography of Dutchess County quality and quantity of groundwater resources, the migration of pollutants, potential hazards to inhabitants, drainage patterns, mineral resources, and soil characteristics. Geology is the study of the earth, including all materials found at and below the earth’s surface. Geologists analyze the composition, origin, and ongoing changes in the rocks and sediments that compose the earth. -
Insights Into the Acadian Orogeny, New England Appalachians: a Provenance Study of the Carrabassett and Kittery Formations, Maine
Insights into the Acadian orogeny, New England Appalachians: a provenance study of the Carrabassett and Kittery formations, Maine Michael J. Dorais1*, Robert P. Wintsch2, Wendy R. Nelson3, and Michael Tubrett4 1. Department of Geological Sciences, Brigham Young University, Provo, Utah 84602, USA 2. Department of Geological Sciences, Indiana University, Bloomington, Indiana 47405, USA 3. Department of Geosciences, Penn State University, University Park, Pennsylvania 16802, USA 4. CREAIT Network, MicroAnalysis Facility, Inco Innovation Centre (MAF-IIC), Memorial University, St. John’s, Newfoundland A1B 3X5, Canada * Corresponding author: <[email protected]> Date received: 07 July 2008 ¶ Date accepted: 11 February 2009 ABSTRACT The Central Maine Basin and Merrimack Trough are Silurian basins that formed adjacent to or were accreted to the Laurentian margin during the Acadian orogeny. The Early Devonian Carrabassett Formation of the Central Maine Basin and the Kittery Formation of the Merrimack Trough have major and trace element compositions indica- tive of a passive continental margin provenance, not unlike the older formations of the Central Maine Basin that are thought to have been derived from Laurentian sources. However, both the Carrabassett and Kittery formations have paleocurrent indicators of outboard sources. The Carrabassett Formation is one of the youngest formations of the Central Maine Basin and was deposited just prior to the Acadian orogeny. The Carrabassett and Kittery formations have major and trace element concentrations suggestive of passive margin turbidites derived from intermediate to felsic sources, inconsistent with a juvenile Avalonian provenance. The Carrabassett Formation contains detrital zircon grains that match the ages of peri-Gondwanan Ganderia. Unlike the dominance of positive bulk-rock εNd values that are characteristic of Avalonia, Ganderia has negative εNd values that are a better match for the negative εNd values of the Carrabassett and Kittery formations. -
Paleozoic Evolution of the Appalachians
Paleozoic Evolution of the Appalachians: Tectonic Overview Three major tectonic episodes, all involving lateral accretion of terranes: deformation, terrane migration, accretion, and continental convergence 1. Ordovician Taconic Orogeny (~470-440 Ma) • collision of Laurentian margin with one or more magmatic arcs Shelburne Falls arc (475-470 Ma) and Bronson Hill arc (454-442 Ma) • or, continent-continent collision between Laurentia and proto-Andean region of Gondwana • slope & rise sediments thrust westward over shelf deposits 2. Devonian Acadian Orogeny (~420-360 Ma) • accretion of Avalon terrane southward continuation of Silurian Caledonian Orogeny (NW Europe) collision of Baltica with Laurentia to form Laurussia • deformation of Bronson Hill arc and sedimentary basins seaward of BH arc at least 3 pulses of deformation • oblique accretion of Avalon and other terranes(?) much strike-slip displacement but also subduction (coastal volcanics) • large mountains erosion creates thick clastic wedge (Catskills and Poccono Mtns.); thinned westward toward cratonic interior 3. Pennsylvansylvanian-Permian Alleghenian Orogeny (~325- 275 Ma) • collision with Gondwanaland consolidation of supercontinent Pangea • extensive zone of deformation New England - Georgia & Alabama (Appalachian Mtns.) - Oklahoma, Arkansas (Ouachita Mtns.) - Texas (Marathon Mtns.) • side-effects: deep crustal shear in Mass., formation of Narragansett rift basin basement block faulting in western interior, uplift of ancestral Rockies "TECTONIC CYCLES" • recorded by the creation of foreland basins sedimentation in eastern New York • associated with tectonic uplift and deformation due to the accretion of island arcs to the east in Massachusetts (first the Ordovician Taconic Orogeny followed by the Devonian Acadian Orogeny: Ordovician Taconic Orogeny (generalized succession in eastern NY) Age Environment Lithology Formation late Ordovician deltaic and molasse Queenston Fm.