Study of the Powerful Nd: YLF Laser Amplifiers for the CTF3 Photoinjectors
Total Page:16
File Type:pdf, Size:1020Kb
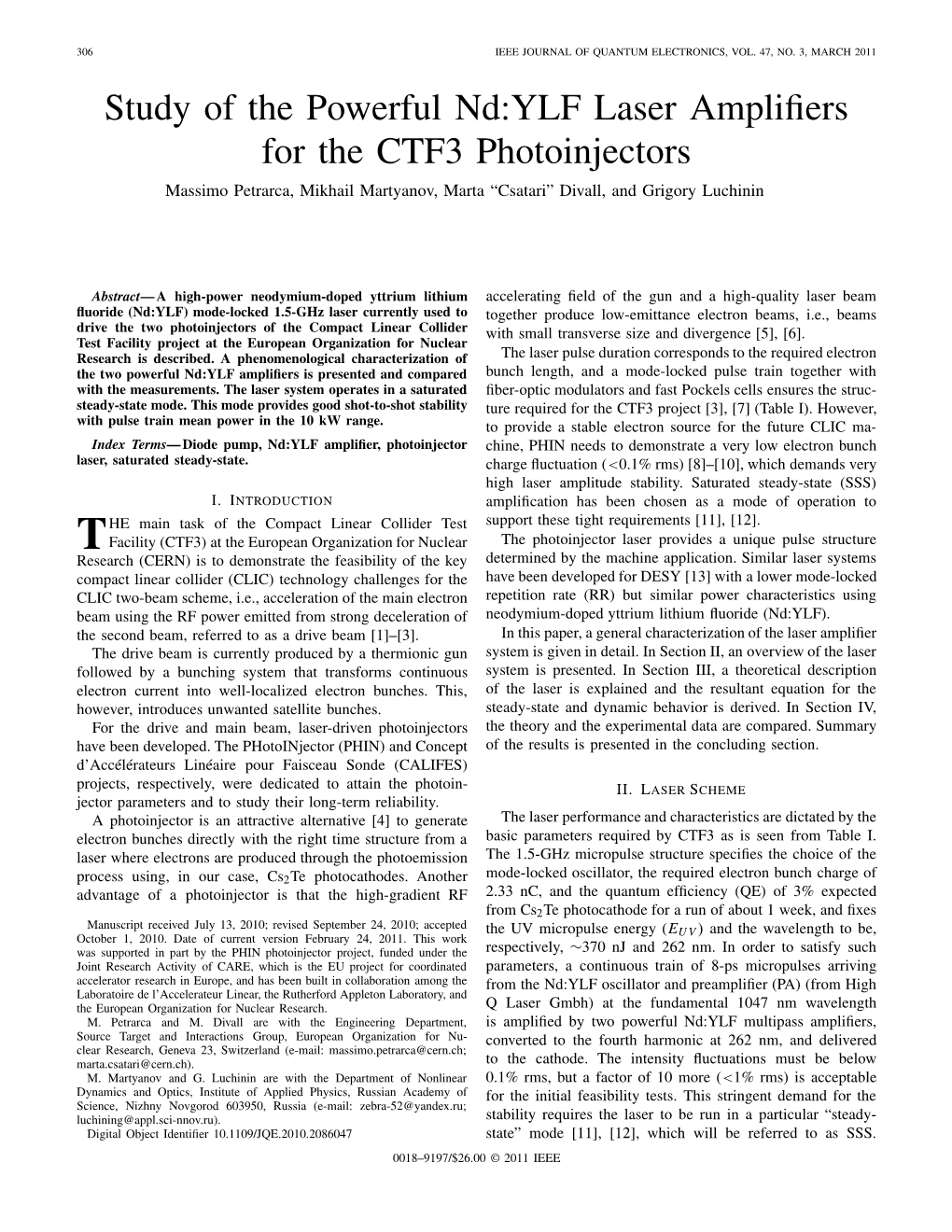
Load more
Recommended publications
-
Light and Color
Light and Color Light is a complex phenomenon that is classically explained with a simple model based on rays and wavefronts. The Molecular Expressions Microscopy Primer explores many of the aspects of visible light starting with an introduction to electromagnetic radiation and continuing through to human vision and the perception of color. Each section outlined below is an independent treatise on a limited aspect of light and color. We hope you enjoy your visit and find the answers to your questions. Electromagnetic Radiation - Visible light is a complex phenomenon that is classically explained with a simple model based on propagating rays and wavefronts, a concept first proposed in the late 1600s by Dutch physicist Christiaan Huygens. Electromagnetic radiation, the larger family of wave-like phenomena to which visible light belongs (also known as radiant energy ), is the primary vehicle transporting energy through the vast reaches of the universe. The mechanisms by which visible light is emitted or absorbed by substances, and how it predictably reacts under varying conditions as it travels through space and the atmosphere, form the basis of the existence of color in our universe. Light: Particle or a Wave? - Many distinguished scientists have attempted to explain how electromagnetic radiation can display what has now been termed duality , or both particle-like and wave-like behavior. At times light behaves as if composed of particles, and at other times as a continuous wave. This complementary, or dual, role for the properties of light can be employed to describe all of the known characteristics that have been observed experimentally, ranging from refraction, reflection, interference, and diffraction, to the results with polarized light and the photoelectric effect. -
Clinical Optics Elkington
Document file:///C|/download/www.netlibrary.com/nlreader/nlreader.dll@bookid=51924&Filename=cover.html9/30/2006 2:34:05 PM Document Page i Clinical Optics file:///C|/download/www.netlibrary.com/nlreader/nlreader.dll@bookid=51924&Filename=Page_I.html9/30/2006 2:34:28 PM Document Page ii Other books of interest Clinical Orthoptics Fiona Rowe 0 632 04274 5 Diagnosis and Management of Ocular Mobility Disorders Second Edition A. Anson & H. Davis 0 632 04798 4 Ocular Anatomy and Physiology T. Saude 0 632 03599 4 Clinical Anatomy of the Eye R.S. Snell & M.A. Lemp 0 632 04344 X Orthoptic Assessment and Management Second Edition D. Stidwill 0 632 05012 8 file:///C|/download/www.netlibrary.com/nlreader/nlreader.dll@bookid=51924&Filename=Page_II.html9/30/2006 2:34:29 PM Document Page iii Clinical Optics Third Edition Andrew R. Elkington CBE, MA, FRCS, FRCOphth Consultant Ophthalmologist Southampton Eye Unit Southampton General Hospital. Professor of Ophthalmology University of Southampton. President, Royal College of Ophthalmologists (1994–1997). Helena J. Frank BMedSci, FRCS, FRCOphth Consultant Ophthalmologist Royal Victoria Hospital Bournemouth. Michael J. Greaney MD, FRCSEd, FRCOphth Specialist Registrar Southampton Eye Unit Southampton General Hospital. file:///C|/download/www.netlibrary.com/nlreader/nlreader.dll@bookid=51924&Filename=Page_III.html (1 of 2)9/30/2006 2:34:30 PM Document Page iv © 1984, 1991, 1999 by Blackwell Science Ltd Editorial Offices: Osney Mead, Oxford OX2 0EL 25 John Street, London WC1N 2BL 23 Ainslie Place, Edinburgh EH3 6AJ 350 Main Street, Malden MA 02148 5018, USA 54 University Street, Carlton Victoria 3053, Australia 10, rue Casimir Delavigne 75006 Paris, France Other Editorial Offices: Blackwell Wissenschafts-Verlag GmbH Kurfürstendamm 57 10707 Berlin, Germany Blackwell Science KK MG Kodenmacho Building 7–10 Kodenmacho Nihombashi Chuo-ku, Tokyo 104, Japan The right of the Author to be identified as the Author of this Work has been asserted in accordance with the Copyright, Designs and Patents Act 1988. -
Global Lithium Sources—Industrial Use and Future in the Electric Vehicle Industry: a Review
resources Review Global Lithium Sources—Industrial Use and Future in the Electric Vehicle Industry: A Review Laurence Kavanagh * , Jerome Keohane, Guiomar Garcia Cabellos, Andrew Lloyd and John Cleary EnviroCORE, Department of Science and Health, Institute of Technology Carlow, Kilkenny, Road, Co., R93-V960 Carlow, Ireland; [email protected] (J.K.); [email protected] (G.G.C.); [email protected] (A.L.); [email protected] (J.C.) * Correspondence: [email protected] Received: 28 July 2018; Accepted: 11 September 2018; Published: 17 September 2018 Abstract: Lithium is a key component in green energy storage technologies and is rapidly becoming a metal of crucial importance to the European Union. The different industrial uses of lithium are discussed in this review along with a compilation of the locations of the main geological sources of lithium. An emphasis is placed on lithium’s use in lithium ion batteries and their use in the electric vehicle industry. The electric vehicle market is driving new demand for lithium resources. The expected scale-up in this sector will put pressure on current lithium supplies. The European Union has a burgeoning demand for lithium and is the second largest consumer of lithium resources. Currently, only 1–2% of worldwide lithium is produced in the European Union (Portugal). There are several lithium mineralisations scattered across Europe, the majority of which are currently undergoing mining feasibility studies. The increasing cost of lithium is driving a new global mining boom and should see many of Europe’s mineralisation’s becoming economic. The information given in this paper is a source of contextual information that can be used to support the European Union’s drive towards a low carbon economy and to develop the field of research. -
X-Ray Spectroscopic Characterization of Laser–Produced Hot Dense Plasmas
THESIS presented at École Polytechnique to obtain the degree of DOCTOR OF ECOLE POLYTECHNIQUE specialization : physics by Nikolaos Kontogiannopoulos Title of the thesis : X-ray spectroscopic characterization of laser–produced hot dense plasmas defended publicly in 6 December 2007 in front of the jury composed of : Jean–Claude Gauthier President Anne Sylvie Gary Referee Jean–Francois Wyart Referee Patrick Audebert Victor Malka Claude Chenais–Popovics Invited Michel Poirier Research Director Acknowledgements This thesis was realized in the laboratory LULI (“Laboratoire pour l’Utilisation des Lasers Intenses”) of Ecole Polytechnique between 2004 and 2007. I would like to thank Francois Amiranoff director of laboratory for providing all the technical and material support and resources for implementing this work. I thank all the members of the jury. Especially Anne Sylvie Gary and Jean–Francois Wyart who carried out the demanding work of being referees. Also, Jean–Claude Gauthier, Patrick Audebert and Victor Malka, who accepted to judge this work. This work had the particular advantage to be accomplished, having as directors Claude Chenais–Popovics and Michel Poirier, which I gratefully thanks for their continuous help in every different problem that I found during my thesis. My gratitude to the members of research group that I had the pleasure to work with : Serena Bastiani–Ceccoti, Jean–Raphael Marques, and Olivier Peurysse. Special thanks to Thomas Blenski the theoretical work of which was a invaluable support in accomplishing my thesis. My grateful thanks to Frederic Thais. Apart from physics, its humor and its positive view gave to me a real reference to continue over the difficulties that I found during this work. -
Review of the Rare Earth Elements and Lithium Mining Sectors
CHEMINFO Review of the Rare Earth Elements and Lithium Mining Sectors FINAL REPORT March 2012 Prepared for: Environment Canada Mining Section Mining and Processing Division Prepared By: Cheminfo Services Inc. 30 Centurian Drive, Suite 205 Markham, Ontario L3R 8B8 Phone: (905) 944-1160 Fax: (905) 944-1175 CHEMINFO Table of Contents 1. EXECUTIVE SUMMARY .....................................................................................1 1.1 INTRODUCTION ....................................................................................................1 1.2 RARE EARTHS .....................................................................................................1 1.2.1 Presence in Canada ...................................................................................5 1.3 LITHIUM ..............................................................................................................5 1.3.1 Presence in Canada ...................................................................................7 1.4 RARE EARTHS AND LITHIUM MINING AND PROCESSING METHODS .....................7 1.4.1 Rare Earth Mining and Processing Methods.............................................7 1.4.2 Lithium Mining and Processing Methods..................................................8 1.5 SUBSTANCES OF ENVIRONMENTAL CONCERN IN RARE EARTH AND LITHIUM MINING AND PROCESSING ...............................................................................................8 1.5.1 Substances of Concern for Rare Earths.....................................................9 -
Design and Commission of the Driven Laser System for Advanced Superconducting Test Accelerator∗
Proceedings of FEL2012, Nara, Japan MOPD56 DESIGN AND COMMISSION OF THE DRIVEN LASER SYSTEM FOR ADVANCED SUPERCONDUCTING TEST ACCELERATOR∗ J. Ruan, J. Santucci, M.Church, Fermi lab, PO Box 500, Batavia, IL 60510, USA Abstract &ŝďĞƌĂƐĞĚƐĞĞĚůĂƐĞƌ ϲϬŵtWƵůƐĞƉŝĐŬĞƌ ϭŵt WƌĞͲĂŵƉ ϭϬŵt DĂŝŶĨŝďĞƌ Currently an advanced superconducting test accelerator ϭ͘ϯ',njƐĞĞĚůĂƐĞƌ ;ϴϭ͘ϮϱD,njͿ ;ϴϭ͘ϮϱD,njͿ ĂŵƉůŝĨŝĞƌ (ASTA) is being built at Fermilab. The accelerator will ϱϬϬŵt consist of an photo electron gun, injector, ILC-type cry- omodules, multiple downstream beam lines for testing cry- ^ŝŶŐůĞƉĂƐƐ Εϯµ: ^ŝŶŐůĞƉĂƐƐ Εϭµ: DW ϲŶ: WƵůƐĞWŝĐŬĞƌ omodules and carrying advanced accelerator research. In ĂŵƉůŝĨŝĞƌ;džϯͿ ƉƵůƐĞ ĂŵƉůŝĨŝĞƌ;džϯͿ ƉƵůƐĞ ĂŵƉůŝĨŝĞƌ ƉƵůƐĞ ;ϯ D,nj Ϳ this paper we will describe the design and commission- Εϵµ: ƉƵůƐĞ ing of the drive laser system for this facility. It consists ^ŝŶŐůĞƉĂƐƐƉŽǁĞƌ ΕϭϴϬµ: ŽƵďůŝŶŐĐƌLJƐƚĂů ΕϴϬµ: YƵĂĚƌŝƉůŝŶŐ ĐƌLJƐƚĂů ΕϮϱµ: of a fiber laser system properly locked to the master fre- ĂŵƉůŝĨŝĞƌ;džϮϬͿ ƉƵůƐĞ ϭϬϱϰͲхϱϮϳŶŵ ƉƵůƐĞ ϱϮϳͲхϮϲϯŶŵ ƉƵůƐĞ quency, a multi-pass amplifier, several power amplifier and &ƌĞĞƐƉĂĐĞĚĂŵƉůŝĨŝĞƌĐŚĂŝŶĂŶĚĨƌĞƋƵĞŶĐLJĐŽŶǀĞƌƐŝŽŶƐƚĂŐĞ final wavelength conversion stage. We will also report the initial characterization of the fiber laser system and the cur- Figure 1: Designed flow chart of the whole photocathode rent commissioning status of the laser system. laser system. The number is the expected power level at each stage.The top box is the fiber based seed laser stage INTRODUCTION and the bottom box is solid state based amplifier chain. A future superconducting RF accelerator test facility is currently being commisioned at Fermilab in the existing pump to the end-pumped active medium has several prac- New Muon Lab (NML) building. -
Spectroscopy and Excitation Dynamics of the Trivalent Lanthanides Tm and Ho in Liyf4
/N- 5- NASA Contractor Report 4689 Spectroscopy and Excitation Dynamics of the Trivalent Lanthanides Tm 3+ and Ho 3+ in LiYF4 Brian M. Walsh Grant NAG1-955 NASA Contractor Report 4689 Spectroscopy and Excitation Dynamics of the Trivalent Lanthanides Tm 3+ and Ho 3+ in LiYF4 Brian M. Walsh Boston College Chestnut Hill, Massachusetts National Aeronautics and Space Administration Prepared for Langley Research Center Langley Research Center Hampton, Virginia 23681-0001 under Grant NAGi -955 August 1995 Printed copies available from the following: NASA Center for Aerospace Information National Technical Information Service (NTIS) 800 EIkridge Landing Road 5285 Port Royal Road Linthicum Heights, MD 21090-2934 Springfield, VA 22161-2171 (301) 621-0390 (703) 487-4650 Dedication This thesis is dedicated to the memory of Clayton H. Bair Spectroscopy and Excitation Dynamics of the Trivalent Lanthanides Tm3+ and H03+ in LiYF4 by Brian M. Walsh Thesis Advisor: Dr. Baldassare Di Bartolo Abstract A detailed study of the spectroscopy and excitation dynamics of the trivalent lanthanides Tm3+ and Ho3+ in Yttrium Lithium Fluoride, LiYF4 (YLF), has been done. YLF is a very versatile laser host that has been used to produce laser action at many different wavelengths when doped with trivalent lanthanides. Since the early 1970's YLF has been the subject of many studies, the main goal of which has been to produce long wavelength lasers in the eye safe 2pm region. This study concentrates on a presentation and analysis of the spectroscopic features, and the temporal evolution of excitation energy in YLF crystals doped with Tm3+ and Ho3+ ions. -
Spectral Analysis and Growth of Nd:YLF Crystal
Asia-Pacific Energy Equipment Engineering Research Conference (AP3ER 2015) Spectral Analysis and Growth of Nd:YLF Crystal Dongyang Zheng, Jialin Xu, Wang Liu, Ximeng Cheng, Weizhao Jin, Chun Li, Fanming Zeng, Hai Lin, Jinghe Liu Changchun University of Science and Technology Changchun , 130022, China e-mail: [email protected] Abstract- communications and other fields.We used a dry method to Nd: YLF polycrystalline raw materials were synthezed by a optimize the process parameters of raw materials ratio and dry method and Nd:YLF laser crystal was grown by IF indu synthesize YLF polycrystalline material. And we used ction Cz method.Its process parameters were these:a pulling medium frequency induction heating pulling method to rate of 1 mm/h, the crystal rotation speed of 15 r/min and 10- 2 grow the Nd:YLF laser crystal, research the spectral Pa degree of vacuum.The absorption and fluorescence spect characteristics of the crystal and analyzed the crystal ra of crystal indicates that the Nd:YLF crystal has strong sbs doped ions in energy level transition. orptions around 808nm at room temperature which belong t o commercial laser diode band and under the 808nm diode la II. EXPERIMENT ser pumped, the fluorescence emission peaks of Nd:YLF crys 4 4 Since the growth of Nd:YLF crystal demanding purity tal are located at 1050and 1300nm ( F3/2→ I11/2) have stronge r emission. raw materials, the chemical reagents LiF, YF3 and NdF3 were selected by the purity of 99.999%.The Keywords-Nd: YLF; Crystal growth; spectral analysis polycrystalline materials were prepared by the dry method. -
Few-Cycle Pulses Amplification for Attosecond Science Applications: Modeling and Experiments
FEW-CYCLE PULSES AMPLIFICATION FOR ATTOSECOND SCIENCE APPLICATIONS: MODELING AND EXPERIMENTS by MICHAËL HEMMER M.S. Engineering Ecole Nationale Supérieure de Physique de Marseille, 2005 A dissertation submitted in partial fulfillment of the requirements for the degree of Doctor of Philosophy in the College of Optics and Photonics at the University of Central Florida Orlando, Florida Spring Term 2011 Major professor: Martin Richardson © 2011 Michaël Hemmer ii ABSTRACT The emergence of mode-locked oscillators providing pulses with durations as short as a few electric-field cycles in the near infra-red has paved the way toward electric-field sensitive physics experiments. In addition, the control of the relative phase between the carrier and the pulse envelope, developed in the early 2000’s and rewarded by a Nobel price in 2005, now provides unprecedented control over the pulse behaviour. The amplification of such pulses to the millijoule level has been an on-going task in a few world-class laboratories and has triggered the dawn of attoscience, the science of events happening on an attosecond timescale. This work describes the theoretical aspects, modeling and experimental implementation of HERACLES, the Laser Plasma Laboratory optical parametric chirped pulse amplifier (OPCPA) designed to deliver amplified carrier-envelope phase stabilized 8-fs pulses with energy beyond 1 mJ at repetition rates up to 10 kHz at 800 nm central wavelength. The design of the hybrid fiber/solid-state amplifier line delivering 85-ps pulses with energy up to 10 mJ at repetition rates in the multi-kHz regime tailored for pumping the optical parametric amplifier stages is presented. -
(12) United States Patent (10) Patent No.: US 7,970,031 B2 Williams Et Al
US00797 0031B2 (12) United States Patent (10) Patent No.: US 7,970,031 B2 Williams et al. (45) Date of Patent: Jun. 28, 2011 (54) Q-SWITCHED LASER WITH PASSIVE (56) References Cited DISCHARGE ASSEMBLY U.S. PATENT DOCUMENTS (75) Inventors: William E. Williams, Melbourne, FL 3,611, 182 A * 10/1971 Treacy ............................ 372/15 3,965,439 A 6, 1976 Firester (US); Charles Carter, Orlando, FL 4,669,085 A 5, 1987 Plourde (US); Robert Pollard, Orlando, FL (US) 4,884,044 A * 1 1/1989 Heywood et al. ............. 359,245 5,384,515 A * 1/1995 Head et al. .................... 313,607 (73) Assignee: FLIR Systems, Inc., Wilsonville, OR RE35,240 E 5/1996 Heywood et al. 5,905,746 A 5/1999 Nguyen et al. (US) 6,501.772 B1 12/2002 Peterson (*) Notice: Subject to any disclaimer, the term of this * cited by examiner patent is extended or adjusted under 35 Primary Examiner — Armando Rodriguez U.S.C. 154(b) by 0 days. (74) Attorney, Agent, or Firm — The H.T. Than Law Group (57) ABSTRACT (21) Appl. No.: 12/616,527 Embodiments of the invention concern a passive discharge assembly comprising one or more Substantially sharp elec (22) Filed: Nov. 11, 2009 trode pins that are positioned proximate to a charged, insu lating Surface. Such as the optical entrance and exit surface of a Q-switch crystal, e.g., lithium niobate (LiNbO). The elec (65) Prior Publication Data trode pins are connected either to the ground or, alternatively, US 2011 FO1 10386 A1 May 12, 2011 to a static source of neutralizing charge. -
The Elements.Pdf
A Periodic Table of the Elements at Los Alamos National Laboratory Los Alamos National Laboratory's Chemistry Division Presents Periodic Table of the Elements A Resource for Elementary, Middle School, and High School Students Click an element for more information: Group** Period 1 18 IA VIIIA 1A 8A 1 2 13 14 15 16 17 2 1 H IIA IIIA IVA VA VIAVIIA He 1.008 2A 3A 4A 5A 6A 7A 4.003 3 4 5 6 7 8 9 10 2 Li Be B C N O F Ne 6.941 9.012 10.81 12.01 14.01 16.00 19.00 20.18 11 12 3 4 5 6 7 8 9 10 11 12 13 14 15 16 17 18 3 Na Mg IIIB IVB VB VIB VIIB ------- VIII IB IIB Al Si P S Cl Ar 22.99 24.31 3B 4B 5B 6B 7B ------- 1B 2B 26.98 28.09 30.97 32.07 35.45 39.95 ------- 8 ------- 19 20 21 22 23 24 25 26 27 28 29 30 31 32 33 34 35 36 4 K Ca Sc Ti V Cr Mn Fe Co Ni Cu Zn Ga Ge As Se Br Kr 39.10 40.08 44.96 47.88 50.94 52.00 54.94 55.85 58.47 58.69 63.55 65.39 69.72 72.59 74.92 78.96 79.90 83.80 37 38 39 40 41 42 43 44 45 46 47 48 49 50 51 52 53 54 5 Rb Sr Y Zr NbMo Tc Ru Rh PdAgCd In Sn Sb Te I Xe 85.47 87.62 88.91 91.22 92.91 95.94 (98) 101.1 102.9 106.4 107.9 112.4 114.8 118.7 121.8 127.6 126.9 131.3 55 56 57 72 73 74 75 76 77 78 79 80 81 82 83 84 85 86 6 Cs Ba La* Hf Ta W Re Os Ir Pt AuHg Tl Pb Bi Po At Rn 132.9 137.3 138.9 178.5 180.9 183.9 186.2 190.2 190.2 195.1 197.0 200.5 204.4 207.2 209.0 (210) (210) (222) 87 88 89 104 105 106 107 108 109 110 111 112 114 116 118 7 Fr Ra Ac~RfDb Sg Bh Hs Mt --- --- --- --- --- --- (223) (226) (227) (257) (260) (263) (262) (265) (266) () () () () () () http://pearl1.lanl.gov/periodic/ (1 of 3) [5/17/2001 4:06:20 PM] A Periodic Table of the Elements at Los Alamos National Laboratory 58 59 60 61 62 63 64 65 66 67 68 69 70 71 Lanthanide Series* Ce Pr NdPmSm Eu Gd TbDyHo Er TmYbLu 140.1 140.9 144.2 (147) 150.4 152.0 157.3 158.9 162.5 164.9 167.3 168.9 173.0 175.0 90 91 92 93 94 95 96 97 98 99 100 101 102 103 Actinide Series~ Th Pa U Np Pu AmCmBk Cf Es FmMdNo Lr 232.0 (231) (238) (237) (242) (243) (247) (247) (249) (254) (253) (256) (254) (257) ** Groups are noted by 3 notation conventions. -
Development of a New Mid-Infrared Source Pumped by an Optical Parametric Chirped-Pulse Amplifier
Development of a new mid-infrared source pumped by an optical parametric chirped-pulse amplifier. by Etienne Pelletier A thesis submitted in conformity with the requirements for the degree of Doctor of Philosophy Graduate Department of Physics University of Toronto Copyright © 2013 by Etienne Pelletier Abstract Development of a new mid-infrared source pumped by an optical parametric chirped-pulse amplifier. Etienne Pelletier Doctor of Philosophy Graduate Department of Physics University of Toronto 2013 The mid-infrared (MIR) system presented in the thesis is based on a sub-100-fs erbium- doped fiber laser operating at 1.55 µm. The output of the laser is split in two, each arm seeding an erbium-doped fiber amplifier. The output of the first amplifier is sent to a grating-based stretcher to be stretched to 50 ps before seeding the optical parametric chirped-pulse amplifier (OPCPA). The output of the second amplifier is coupled to a highly nonlinear fiber to generate the 1 µm needed to seed the a neodymium-doped yttrium lithium fluoride (Nd:YLF) system. This work represents the first time this synchronization scheme is used, and the timing jitter between the two arms at the OPCPA is reduced to 333 fs. The pump laser for the OPCPA is a regenerative amplifier producing 1.6 W followed by a double-pass amplifier, for a final output power of 2.5 W at 1 kHz. Etalons were inserted into the cavity of the regenerative amplifier to stretch the pulses to 50 ps The OPCPA consists of two potassium titanyl arsenate crystals in a noncollinear configuration.