Modeling the Marine Aragonite Cycle: Changes Under Rising Carbon Dioxide and Its Role in Shallow Water Caco3 Dissolution
Total Page:16
File Type:pdf, Size:1020Kb
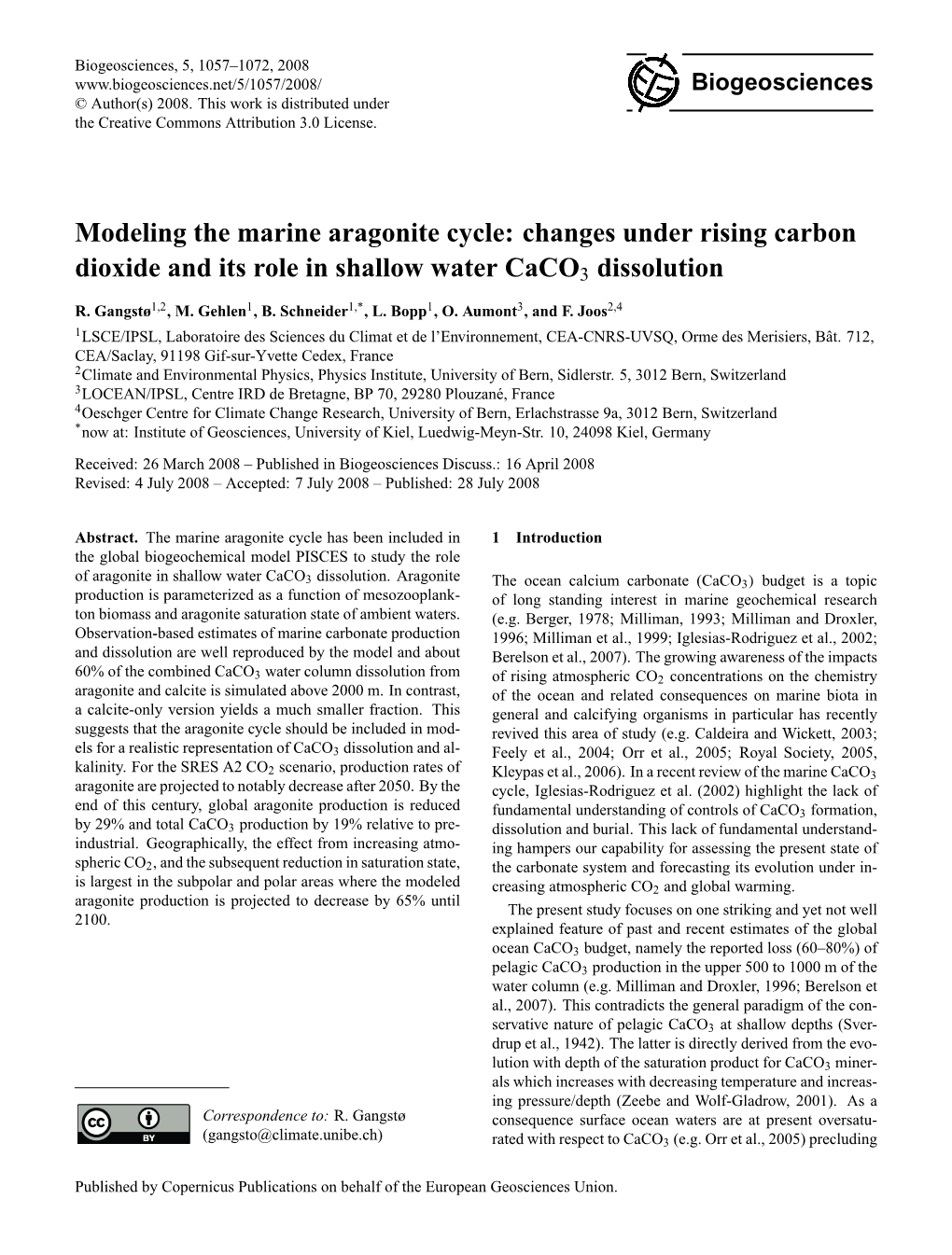
Load more
Recommended publications
-
Coccolith Dissolution Within Copepod Guts Affects Fecal Pellet Density And
www.nature.com/scientificreports OPEN Coccolith dissolution within copepod guts afects fecal pellet density and sinking rate Received: 17 January 2018 Meredith M. White1,2, Jesica D. Waller1, Laura C. Lubelczyk1, David T. Drapeau1, Accepted: 13 June 2018 Bruce C. Bowler1, William M. Balch1 & David M. Fields1 Published: xx xx xxxx The most common biomineral produced in the contemporary ocean is calcium carbonate, including the polymorph calcite produced by coccolithophores. The surface waters of the ocean are supersaturated with respect to calcium carbonate. As a result, particulate inorganic carbon (PIC), such as calcite coccoliths, is not expected thermodynamically to dissolve in waters above the lysocline (~4500– 6000 m). However, observations indicate that up to 60–80% of calcium carbonate is lost in the upper 500–1000 m of the ocean. This is hypothesized to occur in microenvironments with reduced saturation states, such as zooplankton guts. Using a new application of the highly precise 14C microdifusion technique, we show that following a period of starvation, up to 38% of ingested calcite dissolves in copepod guts. After continued feeding, our data show the gut becomes increasingly bufered, which limits further dissolution; this has been termed the Tums hypothesis (after the drugstore remedy for stomach acid). As less calcite dissolves in the gut and is instead egested in fecal pellets, the fecal pellet sinking rates double, with corresponding increases in pellet density. Our results empirically demonstrate that zooplankton guts can facilitate calcite dissolution above the chemical lysocline, and that carbon export through fecal pellet production is variable, based on the feeding history of the copepod. -
Paleoceanographical Proxies Based on Deep-Sea Benthic Foraminiferal Assemblage Characteristics
CHAPTER SEVEN Paleoceanographical Proxies Based on Deep-Sea Benthic Foraminiferal Assemblage Characteristics Frans J. JorissenÃ, Christophe Fontanier and Ellen Thomas Contents 1. Introduction 263 1.1. General introduction 263 1.2. Historical overview of the use of benthic foraminiferal assemblages 266 1.3. Recent advances in benthic foraminiferal ecology 267 2. Benthic Foraminiferal Proxies: A State of the Art 271 2.1. Overview of proxy methods based on benthic foraminiferal assemblage data 271 2.2. Proxies of bottom water oxygenation 273 2.3. Paleoproductivity proxies 285 2.4. The water mass concept 301 2.5. Benthic foraminiferal faunas as indicators of current velocity 304 3. Conclusions 306 Acknowledgements 308 4. Appendix 1 308 References 313 1. Introduction 1.1. General Introduction The most popular proxies based on microfossil assemblage data produce a quanti- tative estimate of a physico-chemical target parameter, usually by applying a transfer function, calibrated on the basis of a large dataset of recent or core-top samples. Examples are planktonic foraminiferal estimates of sea surface temperature (Imbrie & Kipp, 1971) and reconstructions of sea ice coverage based on radiolarian (Lozano & Hays, 1976) or diatom assemblages (Crosta, Pichon, & Burckle, 1998). These à Corresponding author. Developments in Marine Geology, Volume 1 r 2007 Elsevier B.V. ISSN 1572-5480, DOI 10.1016/S1572-5480(07)01012-3 All rights reserved. 263 264 Frans J. Jorissen et al. methods are easy to use, apply empirical relationships that do not require a precise knowledge of the ecology of the organisms, and produce quantitative estimates that can be directly applied to reconstruct paleo-environments, and to test and tune global climate models. -
Soares, R. Et Al
Original Article DOI: 10.7860/JCDR/2017/23594.9758 Assessment of Enamel Remineralisation After Treatment with Four Different Dentistry Section Remineralising Agents: A Scanning Electron Microscopy (SEM) Study RENITA SOARES1, IDA DE NORONHA DE ATAIDE2, MARINA FERNANDES3, RAJAN LAMBOR4 ABSTRACT these groups were remineralised using the four remineralising Introduction: Decades of research has helped to increase our agents. The treated groups were subjected to pH cycling over a knowledge of dental caries and reduce its prevalence. However, period of 30 days. This was followed by assessment of surface according to World Oral Health report, dental caries still remains microhardness and SEM for qualitative evaluation of surface a major dental disease. Fluoride therapy has been utilised in changes. The results were analysed by One-Way Analysis Of a big way to halt caries progression, but has been met with Variance (ANOVA). Multiple comparisons between groups were limitations. This has paved the way for the development of performed by paired t-test and post-hoc Tukey test. newer preventive agents that can function as an adjunct to Results: The results of the study revealed that remineralisation of fluoride or independent of it. enamel was the highest in samples of Group E (Self assembling Aim: The purpose of the present study was to evaluate the ability peptide P11-4) followed by Group B (CPP-ACPF), Group C (BAG) of Casein Phosphopeptide-Amorphous Calcium Phosphate and Group D (fluoride enhanced HA gel). There was a significant Fluoride (CPP ACPF), Bioactive Glass (BAG), fluoride enhanced difference (p<0.05) in the remineralising ability between the self assembling peptide P -4 group and BAG and fluoride Hydroxyapatite (HA) gel and self-assembling peptide P11-4 to 11 remineralise artificial carious lesions in enamel in vitro using enhanced HA gel group. -
Lecture 24 the Oceans Reading: White, Ch 9.1 to 9.7.1 (Or Digital P370-400)
Lecture 24 The Oceans Reading: White, Ch 9.1 to 9.7.1 (or digital p370-400) Last Time 1. The oceans: currents, stratification and chemistry Today 2. the marine carbon cycle GG325 L24, F2013 The Marine Carbon Cycle The marine biosphere and ocean currents control non-conservative chemical signatures in marine water masses. Photosynthetic productivity in the surface waters requires a flux of nutrient- rich waters in to the photic zone. This is variable around the globe. Respirative decomposition of organic matter throughout the marine water column is also variable around the globe. If the oceans did not convect in a semi-steady-state manner, the cycle that controls the concentrations of C, O, N and P in various water masses and the relative biomass that is sustained there would not exist. Today we investigate the movement of carbon in today's oceans. next week we will look to the geologic record to see how past climatic conditions on Earth altered the movement of water masses the motions of carbon through the oceans, plus we consider our future. GG325 L24, F2013 1 Deep Ocean Circulation Effects: The circulation patterns in the 2 oceans that we discussed last time results in what is sometimes referred to as basin-to-basin fractionation. Pacific: deep water inflow is in the south. Return flow is by both upwelling and by diffuse upflow of deep water in the North Pacific. This "in on the bottom out on the top" circulation is like that in most estuaries, thus it is known as estuarine circulation. Atlantic: has deep water formation from This circulation pattern affects nearly every shallow waters: anti- aspect of the marine carbon cycle. -
An Updated Synthesis of the Impacts of Ocean Acidification on Marine Biodiversity 2 3 ACKNOWLEDGEMENTS
P a g e | 1 DRAFT FOR CBD PEER-REVIEW ONLY; NOT TO QUOTE; NOT TO CIRCULATE 1 An updated synthesis of the impacts of ocean acidification on marine biodiversity 2 3 ACKNOWLEDGEMENTS ....................................................................................................... 3 4 5 EXECUTIVE SUMMARY ....................................................................................................... 4 6 7 1. Background and introduction ............................................................................................... 8 8 1.1. Mandate of this review .......................................................................................................... 8 9 1.2. What is ocean acidification? ........................................................................................ 9 10 1.3. Re-visiting key knowledge gaps identified in the previous CBD review ................... 14 11 12 2. Scientific and policy framework ........................................................................................ 17 13 2.1. Steps towards global recognition and international science collaboration ................. 17 14 2.2. Intergovernmental interest in ocean acidification and actions to date ........................ 19 15 16 3. Global status and future trends of ocean acidification ....................................................... 23 17 3.1. Variability .................................................................................................................. 23 18 3.2. Modelled simulations of future ocean -
Aragonite Formation in Confinements: a Step Toward Understanding Polymorph Control COMMENTARY Yifei Xua,B,C and Nico A
COMMENTARY Aragonite formation in confinements: A step toward understanding polymorph control COMMENTARY Yifei Xua,b,c and Nico A. J. M. Sommerdijka,b,c,1 Calcium carbonate (CaCO3) is one of the most com- unclear but were generally attributed to the interac- mon minerals on Earth; it not only forms rocks like tion between the acidic functional groups of the limestone or marble but is also a main component of biomacromolecules and the mineral components. Ad- biominerals such as pearls, the nacre of seashells, and ditionally, it was reported that a macromolecular sea-urchin skeletons (1). Despite many years of re- hydrogel-like 3D network is formed before the miner- search, the polymorphism of CaCO3 is still far from alization of nacre (11), which may play a role in the being understood. CaCO3 has three anhydrous crys- crystallization process by confining the crystallization talline forms: calcite, aragonite, and vaterite, with a to defined small volumes. Nonetheless, confinement decreasing thermodynamic stability under aqueous has never been directly correlated with aragonite for- ambient conditions (calcite > aragonite > vaterite) mation in biominerals, although its capability of con- (2). While vaterite is rare in nature, calcite and arago- trolling crystal orientation and polymorphism has nite are both frequently found in rocks or biominerals been shown in many recent reports (12–15). (1). A well-known example is the aragonite structure of Now, Zeng et al. (5) explore for the first time the nacre (3), where the organization of the crystals leads impact of confinement on aragonite formation. By to extraordinary mechanical performance. However, precipitating CaCO3 within the cylindrical pores of in synthetic systems, crystallization experiments only track-etched membranes (Fig. -
Biotribology Recent Progresses and Future Perspectives
HOSTED BY Available online at www.sciencedirect.com Biosurface and Biotribology ] (]]]]) ]]]–]]] www.elsevier.com/locate/bsbt Biotribology: Recent progresses and future perspectives Z.R. Zhoua,n, Z.M. Jinb,c aSchool of Mechanical Engineering, Southwest Jiaotong University, Chengdu, China bSchool of Mechanical Engineering, Xian Jiaotong University, Xi'an, China cSchool of Mechanical Engineering, University of Leeds, Leeds, UK Received 6 January 2015; received in revised form 3 March 2015; accepted 3 March 2015 Abstract Biotribology deals with all aspects of tribology concerned with biological systems. It is one of the most exciting and rapidly growing areas of tribology. It is recognised as one of the most important considerations in many biological systems as to the understanding of how our natural systems work as well as how diseases are developed and how medical interventions should be applied. Tribological studies associated with biological systems are reviewed in this paper. A brief history, classification as well as current focuses on biotribology research are analysed according to typical papers from selected journals and presentations from a number of important conferences in this area. Progress in joint tribology, skin tribology and oral tribology as well as other representative biological systems is presented. Some remarks are drawn and prospects are discussed. & 2015 Southwest Jiaotong University. Production and hosting by Elsevier B.V. This is an open access article under the CC BY-NC-ND license (http://creativecommons.org/licenses/by-nc-nd/4.0/). Keywords: Biotribology; Biosurface; Joint; Skin; Dental Contents 1. Introduction ...................................................................................2 2. Classifications and focuses of current research. ..........................................................3 3. Joint tribology .................................................................................4 3.1. -
Climate Change and Ocean Acidification Impacts on Lower
EGU Journal Logos (RGB) Open Access Open Access Open Access Advances in Annales Nonlinear Processes Geosciences Geophysicae in Geophysics Open Access Open Access Natural Hazards Natural Hazards and Earth System and Earth System Sciences Sciences Discussions Open Access Open Access Atmospheric Atmospheric Chemistry Chemistry and Physics and Physics Discussions Open Access Open Access Atmospheric Atmospheric Measurement Measurement Techniques Techniques Discussions Open Access Biogeosciences, 10, 5831–5854, 2013 Open Access www.biogeosciences.net/10/5831/2013/ Biogeosciences doi:10.5194/bg-10-5831-2013 Biogeosciences Discussions © Author(s) 2013. CC Attribution 3.0 License. Open Access Open Access Climate Climate of the Past of the Past Discussions Climate change and ocean acidification impacts on lower trophic Open Access Open Access levels and the export of organic carbon to the deepEarth ocean System Earth System Dynamics 1 1 1 2 1 Dynamics A. Yool , E. E. Popova , A. C. Coward , D. Bernie , and T. R. Anderson Discussions 1National Oceanography Centre, University of Southampton Waterfront Campus, European Way, Southampton SO14 3ZH, UK Open Access Open Access 2Met Office Hadley Centre, FitzRoy Road, Exeter EX1 3PB, UK Geoscientific Geoscientific Instrumentation Instrumentation Correspondence to: A. Yool ([email protected]) Methods and Methods and Received: 29 January 2013 – Published in Biogeosciences Discuss.: 25 February 2013 Data Systems Data Systems Revised: 18 July 2013 – Accepted: 19 July 2013 – Published: 5 September 2013 Discussions -
Understanding the Ocean's Biological Carbon Pump in the Past: Do We Have the Right Tools?
Manuscript prepared for Earth-Science Reviews Date: 3 March 2017 Understanding the ocean’s biological carbon pump in the past: Do we have the right tools? Dominik Hülse1, Sandra Arndt1, Jamie D. Wilson1, Guy Munhoven2, and Andy Ridgwell1, 3 1School of Geographical Sciences, University of Bristol, Clifton, Bristol BS8 1SS, UK 2Institute of Astrophysics and Geophysics, University of Liège, B-4000 Liège, Belgium 3Department of Earth Sciences, University of California, Riverside, CA 92521, USA Correspondence to: D. Hülse ([email protected]) Keywords: Biological carbon pump; Earth system models; Ocean biogeochemistry; Marine sedi- ments; Paleoceanography Abstract. The ocean is the biggest carbon reservoir in the surficial carbon cycle and, thus, plays a crucial role in regulating atmospheric CO2 concentrations. Arguably, the most important single com- 5 ponent of the oceanic carbon cycle is the biologically driven sequestration of carbon in both organic and inorganic form- the so-called biological carbon pump. Over the geological past, the intensity of the biological carbon pump has experienced important variability linked to extreme climate events and perturbations of the global carbon cycle. Over the past decades, significant progress has been made in understanding the complex process interplay that controls the intensity of the biological 10 carbon pump. In addition, a number of different paleoclimate modelling tools have been developed and applied to quantitatively explore the biological carbon pump during past climate perturbations and its possible feedbacks on the evolution of the global climate over geological timescales. Here we provide the first, comprehensive overview of the description of the biological carbon pumpin these paleoclimate models with the aim of critically evaluating their ability to represent past marine 15 carbon cycle dynamics. -
Author's Tracked Changes
Toward a global calibration for quantifying past oxygenation in oxygen minimum zones using benthic Foraminifera Martin Tetard1, Laetitia Licari1, Ekaterina Ovsepyan2, Kazuyo Tachikawa1, and Luc Beaufort1 1Aix Marseille Univ, CNRS, IRD, Coll France, INRAE, CEREGE, Aix-en-Provence, France. 2Shirshov Institute of Oceanology, Russian Academy of Sciences, Moscow, Russia. Correspondence: M. Tetard ([email protected]) Abstract. Oxygen Minimum Zones (OMZs) are oceanic areas largely depleted in dissolved oxygen, nowadays considered in expansion in the face of global warming. To investigate the relationship between OMZ expansion and global climate changes during the late Quaternary, quantitative oxygen reconstructions are needed, but are still in their early development. 5 Here, past bottom water oxygenation (BWO) was quantitatively assessed through a new, fast, semi-automated, and taxon-free :::::::::::::::taxon-independent morphometric analysis of benthic foraminiferal tests, developed and calibrated using Eastern North Pacific(ENP ::::WNP::::::::(Western::::::North:::::::Pacific, ::::::::including:::its ::::::::marginal :::::seas), ::::ENP::::::::(Eastern :::::North:::::::Pacific) and the ESP:::::(Eastern South Pa- cific(ESP) OMZs samples. This new approach is based on an average size and circularity index for each sample. This method, as well as two already published micropalaeontological approaches techniques:::::::::based on benthic foraminiferal assemblages 10 variability and porosity investigation of a single species, were here calibrated based on availability of new data from 23 45:: -1 core tops recovered along an oxygen gradient (from 0.03 to 1.79 2.88::::mL.L ) from the ENP, ESP, :::::WNP, ::::ENP,::::EEP::::::::(Eastern ::::::::Equatorial::::::::Pacific), ::::ESP,::::::::SWACM::::::(South:::::West :::::::African ::::::::::Continental :::::::Margin),:AS (Arabian Sea) and WNP (Western North Pacific, including its marginal seas) OMZs. -
Characteristics and Crystal Structure of Calcareous Deposit Films Formed by Electrodeposition Process in Artificial and Natural Seawater
coatings Article Characteristics and Crystal Structure of Calcareous Deposit Films Formed by Electrodeposition Process in Artificial and Natural Seawater Jun-Mu Park 1, Myeong-Hoon Lee 1 and Seung-Hyo Lee 2,* 1 Division of Marine Engineering, Korea Maritime and Ocean University, Busan 49112, Korea; [email protected] (J.-M.P.); [email protected] (M.-H.L.) 2 Department of Ocean Advanced Materials Convergence Engineering, Korea Maritime and Ocean University, Busan 49112, Korea * Correspondence: [email protected] Abstract: In this study, we tried to form the calcareous deposit films by the electrodeposition process. The uniform and compact calcareous deposit films were formed by electrodeposition process and their crystal structure and characteristics were analyzed and evaluated using various surface analytical techniques. The mechanism of formation for the calcareous deposit films could be confirmed and the role of magnesium was verified by experiments in artificial and natural seawater solutions. The highest amount of the calcareous deposit film was obtained at 5 A/m2 while current densities between 1–3 A/m2 facilitated the formation of the most uniform and dense layers. In addition, the adhesion characteristics were found to be the best at 3 A/m2. The excellent characteristics of the calcareous deposit films were obtained when the dense film of brucite-Mg(OH)2 and metastable aragonite-CaCO3 was formed in the appropriate ratio. Citation: Park, J.-M.; Lee, M.-H.; Lee, Keywords: electrodeposition process; calcareous deposit films; aragonite crystal structure; seawater S.-H. Characteristics and Crystal Structure of Calcareous Deposit Films Formed by Electrodeposition Process in Artificial and Natural Seawater. -
Biological Pump
Biological pump The biological pump, in its simplest form, is the ocean's biologically driven sequestration of carbon from the atmosphere to the ocean interior and seafloor sediments.[1] It is the part of the oceanic carbon cycle responsible for the cycling of organic matter formed mainly by phytoplankton during photosynthesis (soft-tissue pump), as well as the cycling of calcium carbonate (CaCO3) formed into shells by certain organisms such as plankton and mollusks (carbonate pump).[2] Air-sea exchange of CO2 Contents Overview Primary production Calcium carbonate Marine snow Quantification Anthropogenic changes See also References Overview The biological pump can be divided into three distinct phases,[4] the first of which is the production of fixed carbon by planktonic phototrophs in the euphotic (sunlit) surface region of the ocean. In these surface waters, phytoplankton use carbon dioxide (CO2), nitrogen (N), phosphorus (P), and other trace elements (barium, iron, zinc, etc.) during photosynthesis to make carbohydrates, lipids, and proteins. Some plankton, (e.g. coccolithophores and foraminifera) combine calcium (Ca) and dissolved carbonates (carbonic acid and bicarbonate) to form a calcium carbonate (CaCO3) protective coating. Once this carbon is fixed into soft or hard tissue, the organisms either stay in the euphotic zone to be recycled as part of the regenerative nutrient cycle or once they die, continue to the second phase of the biological pump and begin to sink to the ocean floor. The sinking particles will often form aggregates as they sink, greatly increasing the sinking rate. It is this aggregation that gives particles a better chance of escaping predation and decomposition in the water column and eventually make it to the sea floor.