Wireless Power Transmission Para Drones.Pdf
Total Page:16
File Type:pdf, Size:1020Kb
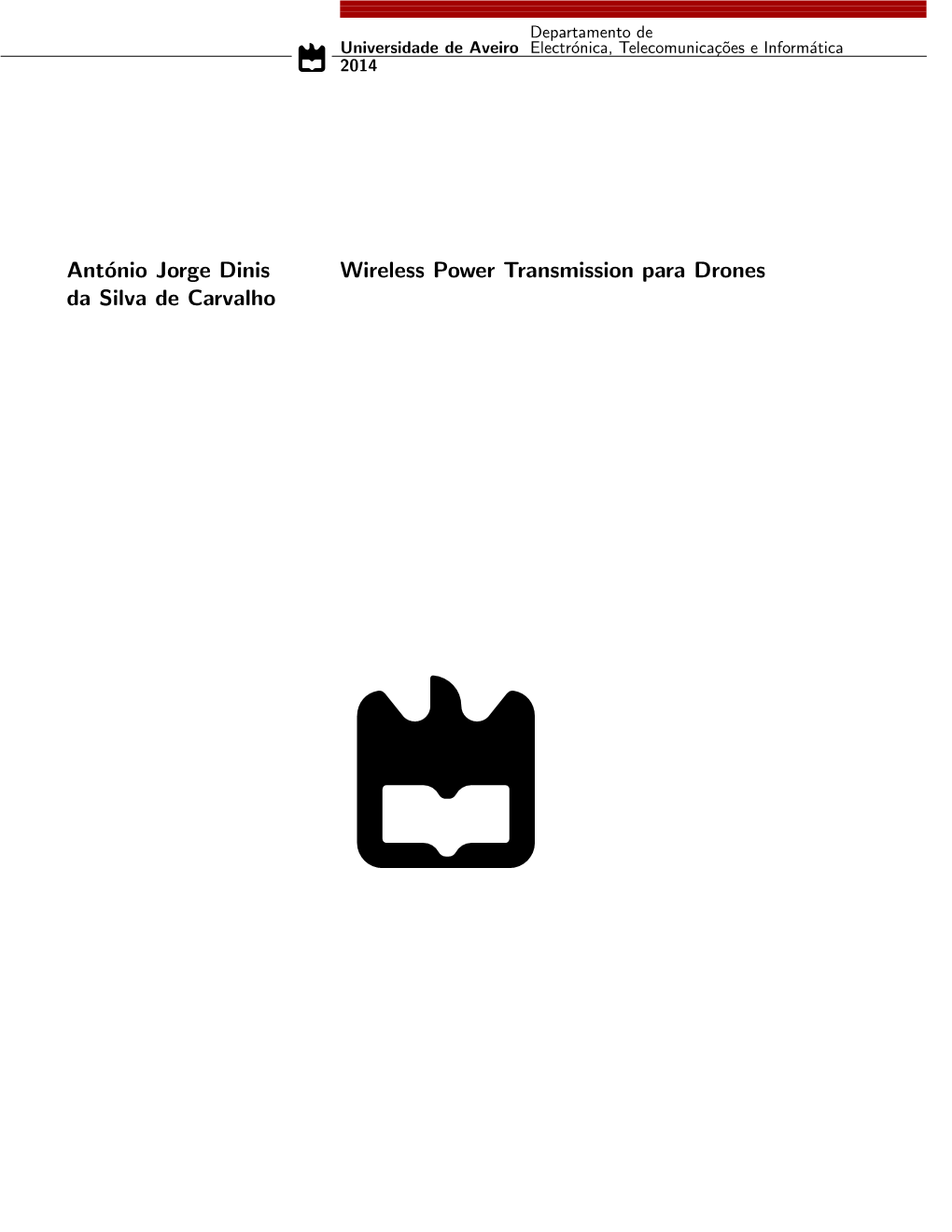
Load more
Recommended publications
-
Nikola Tesla
Nikola Tesla Nikola Tesla Tesla c. 1896 10 July 1856 Born Smiljan, Austrian Empire (modern-day Croatia) 7 January 1943 (aged 86) Died New York City, United States Nikola Tesla Museum, Belgrade, Resting place Serbia Austrian (1856–1891) Citizenship American (1891–1943) Graz University of Technology Education (dropped out) ‹ The template below (Infobox engineering career) is being considered for merging. See templates for discussion to help reach a consensus. › Engineering career Electrical engineering, Discipline Mechanical engineering Alternating current Projects high-voltage, high-frequency power experiments [show] Significant design o [show] Awards o Signature Nikola Tesla (/ˈtɛslə/;[2] Serbo-Croatian: [nǐkola têsla]; Cyrillic: Никола Тесла;[a] 10 July 1856 – 7 January 1943) was a Serbian-American[4][5][6] inventor, electrical engineer, mechanical engineer, and futurist who is best known for his contributions to the design of the modern alternating current (AC) electricity supply system.[7] Born and raised in the Austrian Empire, Tesla studied engineering and physics in the 1870s without receiving a degree, and gained practical experience in the early 1880s working in telephony and at Continental Edison in the new electric power industry. He emigrated in 1884 to the United States, where he became a naturalized citizen. He worked for a short time at the Edison Machine Works in New York City before he struck out on his own. With the help of partners to finance and market his ideas, Tesla set up laboratories and companies in New York to develop a range of electrical and mechanical devices. His alternating current (AC) induction motor and related polyphase AC patents, licensed by Westinghouse Electric in 1888, earned him a considerable amount of money and became the cornerstone of the polyphase system which that company eventually marketed. -
Crowdfunding Is Hot
FEBRUARY 2017 Volume 33 Issue 02 DIGEST Mad Marketing Maven HOW MADMAN MUNTZ BUILT 3 EMPIRES Army Salutes Soldier’s Invention SAVING TIME AND MONEY Big Help for Tiny Babies BUSINESS MODEL: GIVING BACK IT DOESN’T TAKE AN EINSTEIN TO KNOW ... CROWDFUNDING IS HOT $5.95 FULTON, MO FULTON, PERMIT 38 PERMIT US POSTAGE PAID POSTAGE US PRSRT STANDARD PRSRT EDITOR’S NOTE Inventors DIGEST EDITOR-IN-CHIEF REID CREAGER ART DIRECTOR CARRIE BOYD New Website Adds CONTRIBUTORS STAFF SGT. ROBERT R. ADAMS To the Conversation STEVE BRACHMANN DON DEBELAK For many of us, there’s nothing like the traditional magazine experience—hold- JACK LANDER ing the original, glossy physical product in your hands; turning the paper pages; JEREMY LOSAW tearing out portions and/or pages to display or save; and having a flexible, por- GENE QUINN table keepsake that’s never susceptible to electronic or battery failure. JOHN G. RAU A publication that provides both this and a strong internet presence is the best EDIE TOLCHIN of both worlds. With that in mind, Inventors Digest recently updated its website GRAPHIC DESIGNER (inventorsdigest.com). We wanted to make our online content more attractive and JORGE ZEGARRA streamlined, with the goal of inviting even more readers. There’s also more content than ever before; we’re loading more current articles from each issue and going INVENTORS DIGEST LLC back through the archives to pull older ones. This is part of a larger mission: to make PUBLISHER the website a central hub for the Inventors LOUIS FOREMAN Digest community and encourage readers to become active participants in national VICE PRESIDENT, INTERACTIVE AND WEB conversations involving invented-related MATT SPANGARD subjects. -
Tesla's Magnifying Transmitter Principles of Working
School of Electrical Engineering University of Belgrade Tesla's magnifying transmitter principles of working Dr Jovan Cvetić, full prof. School of Electrical Engineering Belgrade, Serbia [email protected] School of Electrical Engineering University of Belgrade Contens The development of the HF, HV generators with oscilatory (LC) circuits: I - Tesla transformer (two weakly coupled LC circuits, the energy of a single charge in the primary capacitor transforms in the energy stored in the capacitance of the secondary, used by Tesla before 1891). It generates the damped oscillations. Since the coils are treated as the lumped elements the condition that the length of the wire of the secondary coil = quarter the wave length has a small impact on the magnitude of the induced voltage on the secondary. Using this method the maximum secondary voltage reaches about 10 MV with the frequency smaller than 50 kHz. II – The transformer with an extra coil (Magnifying transformer, Colorado Springs, 1899/1900). The extra coil is treated as a wave guide. Therefore the condition that the length of the wire of the secondary coil = quarter the wave length (or a little less) is necessary for the correct functioning of the coil. The purpose of using the extra coil is the generation of continuous harmonic oscillations of the great magnitude (theoretically infinite if there are no losses). The energy of many single charges in the primary capacitor are synchronously transferred to the extra coil enlarging the magnitude of the oscillations of the stationary wave in the coil. The maximum voltage is limited only by the breakdown voltage of the insulation on the top of the extra coil and by its dimensions. -
Animation! [Page 8–9] 772535 293004 the TOWER 9> Playing in the Online Dark
9 euro | SPRING 2020 MODERN TIMES REVIEW THE EUROPEAN DOCUMENTARY MAGAZINE CPH:DOX THESSALONIKI DF ONE WORLD CINÉMA DU RÉEL Copenhagen, Denmark Thessaloniki, Greece Prague, Czech Paris, France Intellectually stimulating and emotionally engaging? [page 10–11] THE PAINTER AND THE THIEF THE PAINTER HUMAN IDFF MAJORDOCS BOOKS PHOTOGRAPHY Oslo, Norway Palma, Mallorca New Big Tech, New Left Cinema The Self Portrait; Dear Mr. Picasso Animation! [page 8–9] 772535 293004 THE TOWER 9> Playing in the online dark In its more halcyon early days, nature of these interactions. ABUSE: In a radical the internet was welcomed Still, the messages and shared psychosocial experiment, into households for its utopi- (albeit blurred for us) images an possibilities. A constantly are highly disturbing, the bra- the scope of online updating trove of searchable zenness and sheer volume of child abuse in the Czech information made bound en- the approaches enough to sha- Republic is uncovered. cyclopaedia sets all but obso- ke anyone’s trust in basic hu- lete; email and social media manity to the core («potential- BY CARMEN GRAY promised to connect citizens ly triggering» is a word applied of the world, no longer seg- to films liberally these days, Caught in the Net mented into tribes by physical but if any film warrants it, it is distance, in greater cultural un- surely this one). Director Vit Klusák, Barbora derstanding. The make-up artist recog- Chalupová In the rush of enthusiasm, nises one of the men and is Czech Republic, Slovakia the old truth was suspended, chilled to witness this behav- that tools are only as enlight- iour from someone she knows, ened as their users. -
Wireless Power Transmission
IOSR Journal of Electrical and Electronics Engineering (IOSR-JEEE) e-ISSN: 2278-1676,p-ISSN: 2320-3331, Volume 9, Issue 3 Ver. II (May – Jun. 2014), PP 01-05 www.iosrjournals.org Wireless Power Transmission 1Sudha Bhutada(Senior Lect.), 2Punit Maheshwari(R&D Eng.) 1EE Dept.SV Polytechnic College Bhopal, India 2VLSI Dept. Navigator Technologies Bhopal, India Abstract: The concept of wireless power transmission method is as old as we are using electricity. N. Tesla the father of wireless power transmission was the first to give an idea of wireless power transmission and he was keenly interested in lightning the world without using wires (WPT).Since then series of experiments were made to make feasible transmission of power where it is not possible and commercially viable by advances, inventions in electronic devices in this field. In this paper we present the concept of wireless power transmission from one place in turn to another. This concept is mainly used for reducing the transmission and distribution losses. This in turn reduces the cost of transmission and distribution and also the cost of electrical energy for the consumers. In this paper we discussed the methods, recent developments in WPT, its merits, demerits and applications. Keywords: Wireless Power Transmission WPT, Radio Frequency RF I. Introduction We know our present system of overhead transmission and distribution has biggest disadvantage is percentage of power loss is around 26 to 28 % during transmission and distribution which is because of resistance of wires of grid. WRI (World resources Institute) says that India's electricity grid has highest T&D losses in the world around 27%. -
Download [1.94
The essential Nikola Tesla: peacebuilding endeavor © 2015 Tesla Memory Project, UNESCO Center for Peace. All rights reserved. No part of this publication may be reproduced, stored in a retrieval system or transmitted in any form or by any means, without the prior permission of the publisher Published by Tesla Memory Project UNESCO Center for Peace TESLIANUM Energy Innovation Center Editorial board Dragoljub Martinović Mirjana Prljević Srdjan Pavlović Guy Djoken Editor in chief Aleksandar Protić We appreciate the help of Peace and Crisis Management Foundation for their precious help in publishing The essential Nikola Tesla : peacebuilding endeavor CONTENTS INTRODUCTION 17 Robert Curl: Tesla’s unique place in the history of invention 21 Gordana Vunjak- Novaković: Nikola Tesla - a pure genius and ultimate humanitarian 25 Guy Djoken: Nikola Tesla, Advocate for the Human Condition and Martyr for a greedless society 30 Mirjana Prljević: Tesla, the world strategist 34 Dragoljub Martinović: Wonderful Tesla – inspiration and guidepost 38 Jean Echenoz: Character of an erudite 42 Zorica Civrić: Tesla and Shakespeare: Similarities of genius and heroic drama 44 Ranko Rajović, Uroš Petrović: Nikola Tesla as the inspiration to establish a program for development of children’s integral potentials 49 Yves Lopez: Nikola Tesla inspiring UNESCO 55 Natasha Cica: THINKtent Tesla 59 Romilo Knežević: Tesla - Scientist, “Homo Theurgos” and Saint 63 Aleksandra Ninković-Tašić: Michael Pupin and Nikola Tesla: new perspective on the history of science 67 Rave Mehta: -
Extracting Microrna-Gene Relations from Biomedical Literature Using Distant Supervision
RESEARCH ARTICLE Extracting microRNA-gene relations from biomedical literature using distant supervision Andre Lamurias1*, Luka A. Clarke2, Francisco M. Couto1 1 LaSIGE, Faculdade de Ciências, Universidade de Lisboa, Lisboa, Portugal, 2 BioISI: Biosystems & Integrative Sciences Institute, Faculdade de Ciências, Universidade de Lisboa, Lisboa, Portugal * [email protected] a1111111111 a1111111111 a1111111111 Abstract a1111111111 a1111111111 Many biomedical relation extraction approaches are based on supervised machine learning, requiring an annotated corpus. Distant supervision aims at training a classifier by combining a knowledge base with a corpus, reducing the amount of manual effort necessary. This is particularly useful for biomedicine because many databases and ontologies have been made available for many biological processes, while the availability of annotated corpora is OPEN ACCESS still limited. We studied the extraction of microRNA-gene relations from text. MicroRNA reg- Citation: Lamurias A, Clarke LA, Couto FM (2017) Extracting microRNA-gene relations from ulation is an important biological process due to its close association with human diseases. biomedical literature using distant supervision. The proposed method, IBRel, is based on distantly supervised multi-instance learning. We PLoS ONE 12(3): e0171929. doi:10.1371/journal. evaluated IBRel on three datasets, and the results were compared with a co-occurrence pone.0171929 approach as well as a supervised machine learning algorithm. While supervised learning Editor: Quan Zou, Tianjin University, CHINA outperformed on two of those datasets, IBRel obtained an F-score 28.3 percentage points Received: September 22, 2016 higher on the dataset for which there was no training set developed specifically. To demon- Accepted: January 29, 2017 strate the applicability of IBRel, we used it to extract 27 miRNA-gene relations from recently published papers about cystic fibrosis. -
The Fight to Preserve Tesla's Wardenclyffe Laboratory
The Fight to Preserve Tesla’s Wardenclyffe Laboratory Marianne Macy Excerpt from Margaret Cheney’s Tesla: Man Out of Time (Barnes and Noble Books, 1981, pp. 173-175) The world system for broadcasting—a concept designed to incorporate almost every aspect of modern communications—was all over but the mourning. Yet as long as the tower stood, Tesla continued his efforts to complete it. Exactly when all the workers left, no one could say. Thomas R. Bayles, the general passenger agent of the railroad station just across the road from the abandoned plant, only noticed that passengers had stopped getting off there. A caretaker remained on duty for a time. When curi- ous journalists or research engineers showed up they were allowed to climb to the tower top with the sweeping view of Long Island Sound. For all that the tower looked so light, it was built entirely without metal, even down to the wooden pegs holding together the wooden uprights and cross members. After abandoning the plan for covering the dome with a copper sheathing, Tesla had installed a removable disk through which a beam of radiation could be projected to the zenith. The visitors found the laboratory filled with curiously complex apparatus. In addition to much glass-blowing equipment there were a com- plex machine shop with eight lathes, X-ray devices, a great variety of high-frequency Tesla coils, one of his original radio-controlled robot boats and exhibit cases filled with thousands of bulbs and tubes. There were an office, library, instrument room, electrical generators and transform- ers, and great stocks of wire and cable. -
Wireless Transmission of Electrical Power Overview of Recent Research & Development
International Journal of Computer and Electrical Engineering, Vol.4, No.2, April 2012 Wireless Transmission of Electrical Power Overview of Recent Research & Development Sagolsem Kripachariya Singh, T. S. Hasarmani, and R. M. Holmukhe wireless transmission at 1891 in his “experimental Abstract— The aim of this research work is to give a overview station” at Colorado. Nikola Tesla successfully lighted a of recent researches and development in the field of wireless small incandescent lamp by means of a resonant circuit power transmission. The methods applied for wireless power grounded on one end. A coil outside laboratory with the transmission like Induction, Electromagnetic transmission, Evanescent wave coupling, Electrodynamic induction, Radio lower end connected to the ground and the upper end free. and microwave and Electrostatic Induction ,are discussed.This The lamp is lighted by the current induced in the three study also focuses on the latest technologies, merits and demerits turns of wire wound around the lower end of the coil. in this field. The economic aspects are briefly discussed. Index Terms—wireless transmission, witricity, power, solarpower I. INTRODUCTION In the past, product designers and engineers have faced challenges involving power: the continuity of supplied power, recharging batteries, optimizing the location of sensors, and dealing with rotating or moving joints. Although those Fig. 1. Tesla’s experimental lamp. challenges remain, new demands that arise from increased use of mobile devices and operation in dirty or wet environments mean that designers require new approaches to supplying power to equipment.Wireless Power Transmission from the time of Tesla has been an underdeveloped technology. Tesla had always tried to introduce worldwide wireless power distribution system. -
Abstract Over the Past Few Decades, the Methods of Electrical Generation
Abstract Over the past few decades, the methods of electrical generation such as by coal, nuclear fission, solar, wind, and geothermal have been studied and tested repeatedly, yet the mode of electrical transmission has remained the same: An electric grid of wires. However, at the turn of the 20th century, inventor and electrical pioneer Nikola Tesla attempted to build the Wardenclyffe Tower, a giant Tesla coil which he claimed could transmit power over large distances using induction. Unfortunately, funding for his research dried up and his claims have been dismissed. If Tesla's claims are true, the land and resource-consuming power grid can be eliminated. The magnitude of Tesla's vision was not something we attempted to test physically; rather, we intended to apply the results of small-scale transmission from a Tesla coil to the large scale to measure its feasibility. For our tests, we built a Tesla coil and measured its power usage. Next, we measured the power of a fluorescent light bulb and the distance from the coil at which it glowed. Finally, we compared the power and costs of our Tesla coil to that of North Anna's nuclear facilities, which we used as an example power station. Our results matched that of previous studies - the power output from a Tesla coil decreases dramatically with distance and thus would not be feasible as an efficient power transmitter. Although further research is needed on wireless effects on the large scale, the current electric grid remains the most efficient power transmission. Question In the late 19th century, Edison’s mode of electrical transfer using wires, won out over Tesla’s dream of wireless electricity. -
Wireless Power Transfer (WPT) Via Magnetic Induction Is an Emerging Technology That Is a Result of the Significant Advancements in Power Electronics
CRANFIELD UNIVERSITY Samer Aldhaher Design and Optimization of Switched-Mode Circuits for Inductive Links School of Engineering Energy & Power Engineering Division PhD Thesis Academic Year: 2010 - 2014 Supervisor: Prof. Patrick Chi-Kwong Luk January 2014 ii CRANFIELD UNIVERSITY School of Engineering Energy & Power Engineering Division PhD THESIS Academic Year 2010 - 2014 Samer Aldhaher DESIGN AND OPTIMIZATION OF SWITCHED-MODE CIRCUITS FOR INDUCTIVE LINKS Supervisor: Prof. Patrick Chi-Kwong Luk January 2014 This thesis is submitted in partial fulfilment of the requirements for the degree of Doctor of Philosophy © Cranfield University 2014. All rights reserved. No part of this publication may be reproduced without the written permission of the copyright owner. iv to my parents vi Abstract Wireless power transfer (WPT) via magnetic induction is an emerging technology that is a result of the significant advancements in power electronics. Mobiles phones can now be charged wirelessly by placing them on a charging surface. Electric vehicles can charge their batteries while being parked over a certain charging spot. The possible applications of this technology are vast and the potential it has to revolutionise and change the way that we use today’s application is huge. Wireless power transfer via magnetic induction, also referred to as inductive power transfer (IPT), does not necessarily aim to replace the cable. It is intended to coexist and operate in conjunction with the cable. Although significant progress has been achieved, it is still far from reaching this aim since many obstacles and design chal- lenges still need to be addressed. Low power efficiencies and limited transfer range are the two main issues for IPT. -
Wireless Energy Transfer Using Resonant Magnetic Induction for Electric Vehicle Charging Application Neelima Dahal Clemson University, [email protected]
Clemson University TigerPrints All Theses Theses 5-2017 Wireless Energy Transfer Using Resonant Magnetic Induction for Electric Vehicle Charging Application Neelima Dahal Clemson University, [email protected] Follow this and additional works at: https://tigerprints.clemson.edu/all_theses Recommended Citation Dahal, Neelima, "Wireless Energy Transfer Using Resonant Magnetic Induction for Electric Vehicle Charging Application" (2017). All Theses. 2614. https://tigerprints.clemson.edu/all_theses/2614 This Thesis is brought to you for free and open access by the Theses at TigerPrints. It has been accepted for inclusion in All Theses by an authorized administrator of TigerPrints. For more information, please contact [email protected]. WIRELESS ENERGY TRANSFER USING RESONANT MAGNETIC INDUCTION FOR ELECTRIC VEHICLE CHARGING APPLICATION A Thesis Presented to the Graduate School of Clemson University In Partial Fulfillment of the Requirements for the Degree Master of Science Electrical Engineering by Neelima Dahal May 2017 Accepted by: Dr. Anthony Q. Martin, Committee Chair Dr. Pingshan Wang Dr. Harlan B. Russell ABSTRACT The research work for this thesis is based on utilizing resonant magnetic induction for wirelessly charging electric vehicles. The background theory for electromagnetic induction between two conducting loops is given and it is shown that an RLC equivalent circuit can be used to model the loops. An analysis of the equivalent circuit is used to show how two loosely coupled loops can be made to exchange energy efficiently by operating them at a frequency which is the same as the resonant frequency of both. Furthermore, it is shown that the efficiency is the maximum for critical coupling (determined by the quality factors of the loops), and increasing the coupling beyond critical coupling causes double humps to appear in the transmission efficiency versus frequency spectrum.