Onion Root Anatomy and the Uptake of Sulphate and Phosphate Ions
Total Page:16
File Type:pdf, Size:1020Kb
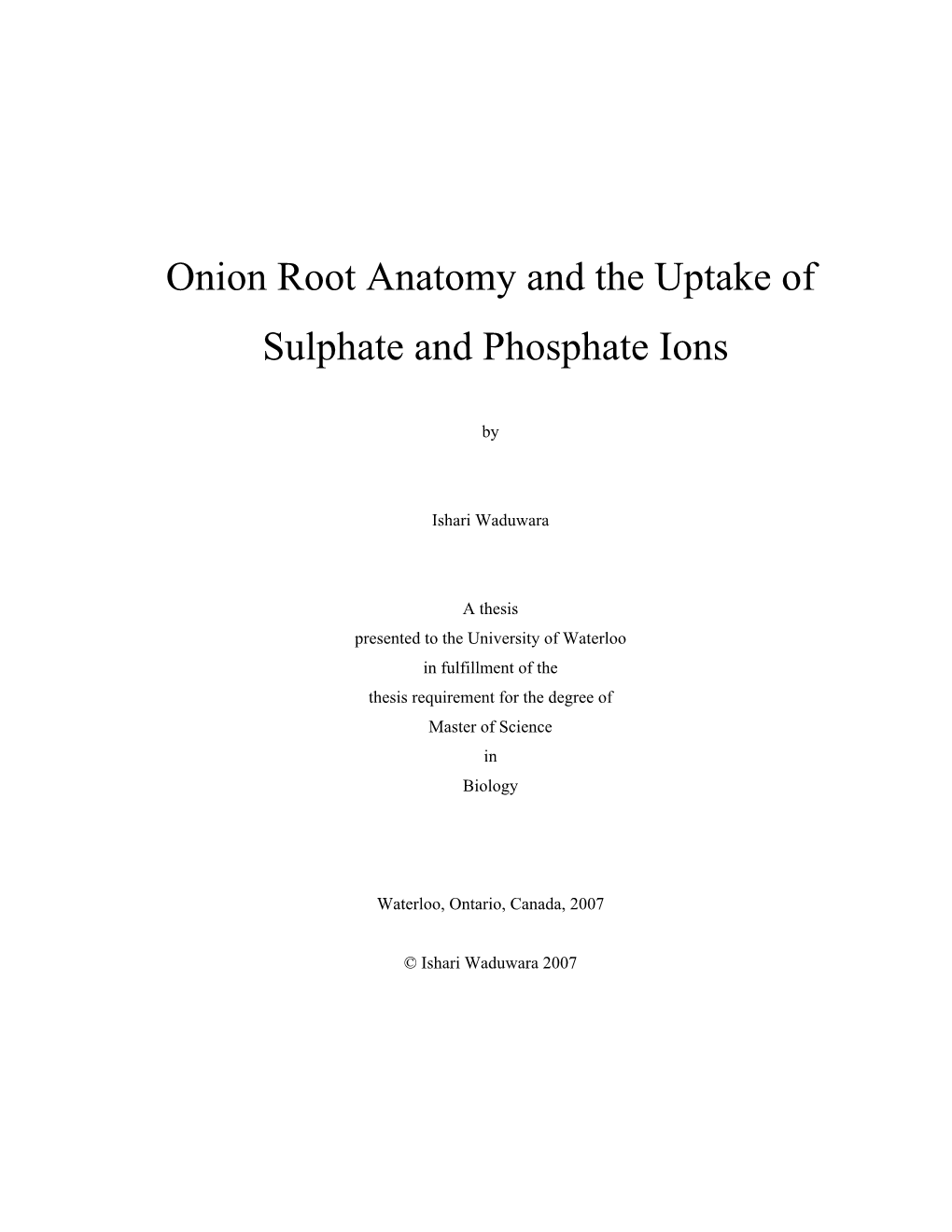
Load more
Recommended publications
-
Differentiation of Epidermis with Reference to Stomata
Unit : 3 Differentiation of Epidermis with Reference to Stomata LESSON STRUCTURE 3.0 Objective 3.1 Introduction 3.2 Epidermis : Basic concept, its function, origin and structure 3.3 Stomatal distribution, development and classification. 3.4 Questions for Exercise 3.5 Suggested Readings 3.0 OBJECTIVE The epidermis, being superficial or outermost layer of cells, covers the entire plant body. It includes structures like stomata and trichomes. Distribution of stomata in epidermis depends on Ontogeny, number of subsidiary cells, separation of guard cells and different taxonomic ranks (classes, families and species). 3.1 INTRODUCTION The internal organs of plants are covered by a well developed tissue system, the epidermal or integumentary system. The epidermis modifies itself to cope up with natural surroundings, since, it is in direct contact with the environment. It protects the inner tissues from any adverse natural calamities like high temperature, desiccation, mechanical injury, excessive illumination, external infection etc. In some plants epidermis may persist throughout the life, while in others it is replaced by periderm. Although the epiderm usually arises from the outer most tunica layer, which thus coincides with Hanstein’s dermatogen, the underlying tissues may have their origin in the tunica or the corpus or both, depending on plant species and the number of tunica layers, explained by Schmidt (1924). ( 38 ) Differentiation of Epidermis with Reference to Stomata 3.2 EPIDERMIS Basic Concept The term epidermis designates the outer most layer of cells on the primary plant body. The word is derived from two Greek words ‘epi’ means upon and ‘derma’ means skin. Through the history of development of plant morphology the concept of the epidermis has undergone changes, and there is still no complete uniformity in the application of the term. -
Stoma and Peristomal Skin Care: a Clinical Review Early Intervention in Managing Complications Is Key
WOUND WISE 1.5 HOURS CE Continuing Education A series on wound care in collaboration with the World Council of Enterostomal Therapists Stoma and Peristomal Skin Care: A Clinical Review Early intervention in managing complications is key. ABSTRACT: Nursing students who don’t specialize in ostomy care typically gain limited experience in the care of patients with fecal or urinary stomas. This lack of experience often leads to a lack of confidence when nurses care for these patients. Also, stoma care resources are not always readily available to the nurse, and not all hospitals employ nurses who specialize in wound, ostomy, and continence (WOC) nursing. Those that do employ WOC nurses usually don’t schedule them 24 hours a day, seven days a week. The aim of this article is to provide information about stomas and their complications to nurses who are not ostomy specialists. This article covers the appearance of a normal stoma, early postoperative stoma complications, and later complications of the stoma and peristomal skin. Keywords: complications, ostomy, peristomal skin, stoma n 46 years of clinical practice, I’ve encountered article covers essential information about stomas, many nurses who reported having little educa- stoma complications, and peristomal skin problems. Ition and even less clinical experience with pa- It is intended to be a brief overview; it doesn’t provide tients who have fecal or urinary stomas. These exhaustive information on the management of com- nurses have said that when they encounter a pa- plications, nor does it replace the need for consulta- tient who has had an ostomy, they are often un- tion with a qualified wound, ostomy, and continence sure how to care for the stoma and how to assess (WOC) nurse. -
Book of Abstracts
CHALLENGES FOR PLANT NUTRITION IN CHANGING ENVIRONMENTS International Workshop and Meeting of the German Society of Plant Nutrition 2012 University of Bonn September 5 – 8, 2012 Book of Abstracts Table of Contents Plenary Session: Introductory talks ................................................. 2 Plenary Session S1: Processes on leaf surfaces ................................. 5 Plenary Session S2: Plant water relations .......................................... 14 Plenary Session S3: Nutrient dynamics in changing environments .... 26 Plenary Session S4: Crop responses to nutrient imbalances ............. 38 Plenary Session S5: Phenotyping and early stress responses ........... 80 Poster Session P1: Fertilization (inorganic) ...................................... 92 Poster Session P2: Fertilization (organic) / Soil amendments ........ 110 Poster Session P3: Nutrient efficiency / Genomics ......................... 131 Poster Session P4: Root physiology / Root-soil interactions........... 148 Poster Session P5: Physiological response to abiotic stress .......... 169 Poster Session P6: Physiological response to nutrient imbalances 182 Poster Session P7: Nutrients and ecosystems / Climate change ... 200 Poster Session P8: Signalling / Quality / Phenotyping .................... 215 1 DGP Meeting September 5-9, 2012 Plenary Session: Introductory talks 2 DGP Meeting September 5-9, 2012 Plant nutrition in a changing environment. Patrick H. Brown Department of Plant Sciences, University of California, Davis, CA 95616, US; E-mail: [email protected] The scientific discipline of plant nutrition is wonderfully broad in its scale and its scope. From the exploration of the function of nutrients as signals and regulators of plant function, to the role of plant nutrients in agricultural productivity and food quality, to the exploration of the effects of nutrient losses on global environments, plant nutrition is a truly integrative discipline and will play a critical role in mans’ ability to adapt to environmental change. -
Development and Cell Cycle Activity of the Root Apical Meristem in the Fern Ceratopteris Richardii
G C A T T A C G G C A T genes Article Development and Cell Cycle Activity of the Root Apical Meristem in the Fern Ceratopteris richardii Alejandro Aragón-Raygoza 1,2 , Alejandra Vasco 3, Ikram Blilou 4, Luis Herrera-Estrella 2,5 and Alfredo Cruz-Ramírez 1,* 1 Molecular and Developmental Complexity Group at Unidad de Genómica Avanzada, Laboratorio Nacional de Genómica para la Biodiversidad, Cinvestav Sede Irapuato, Km. 9.6 Libramiento Norte Carretera, Irapuato-León, Irapuato 36821, Guanajuato, Mexico; [email protected] 2 Metabolic Engineering Group, Unidad de Genómica Avanzada, Laboratorio Nacional de Genómica para la Biodiversidad, Cinvestav Sede Irapuato, Km. 9.6 Libramiento Norte Carretera, Irapuato-León, Irapuato 36821, Guanajuato, Mexico; [email protected] 3 Botanical Research Institute of Texas (BRIT), Fort Worth, TX 76107-3400, USA; [email protected] 4 Laboratory of Plant Cell and Developmental Biology, Division of Biological and Environmental Sciences and Engineering (BESE), King Abdullah University of Science and Technology (KAUST), Thuwal 23955-6900, Saudi Arabia; [email protected] 5 Institute of Genomics for Crop Abiotic Stress Tolerance, Department of Plant and Soil Science, Texas Tech University, Lubbock, TX 79409, USA * Correspondence: [email protected] Received: 27 October 2020; Accepted: 26 November 2020; Published: 4 December 2020 Abstract: Ferns are a representative clade in plant evolution although underestimated in the genomic era. Ceratopteris richardii is an emergent model for developmental processes in ferns, yet a complete scheme of the different growth stages is necessary. Here, we present a developmental analysis, at the tissue and cellular levels, of the first shoot-borne root of Ceratopteris. -
The Genomics of Wood Formation in Angiosperm Trees
The Genomics of Wood Formation in Angiosperm Trees Xinqiang He and Andrew T. Groover Abstract Advances in genomic science have enabled comparative approaches that can evaluate the evolution of genes and mechanisms underlying phenotypic traits relevant to angiosperm forest trees. Wood formation is an excellent subject for com- parative genomics, as it is an ancestral trait for angiosperms and has undergone significant modification in different angiosperm lineages. This chapter discusses some of the traits associated with wood formation, what is currently known about the genes and mechanisms regulating these traits, and how comparative evolution- ary genomic studies can be undertaken to provide more comprehensive views of the evolution and development of wood formation in angiosperms. Keywords Wood formation • Wood development • Wood evolution • Transcriptional regulation • Epigenetics • Comparative genomics Genomic Perspectives on the Evolutionary Origins and Variation in Angiosperm Wood Introduction The evolutionary and developmental biology of wood are fundamental to under- standing the amazing diversification of angiosperms. While flower morphology and reproductive characters associated with angiosperms have been the focus of numer- ous genetic and genomic evo-devo studies, wood development is a relatively neglected trait of angiosperm evolution and development that is now tractable for comparative and evolutionary genomic studies. The ability to produce wood from a X. He School of Life Sciences, Peking University, Beijing 100871, China A.T. Groover (*) Pacific Southwest Research Station, US Forest Service, Davis, 95618 CA, USA Department of Plant Biology, University of California Davis, Davis, 95618 CA, USA e-mail: [email protected] © Springer International Publishing AG 2017 205 A.T. Groover and Q.C.B. -
Testing the Mьnch Hypothesis of Long Distance Phloem Transport in Plants
Downloaded from orbit.dtu.dk on: Oct 10, 2021 Testing the Münch hypothesis of long distance phloem transport in plants Knoblauch, Michael; Knoblauch, Jan; Mullendore, Daniel L.; Savage, Jessica A.; Babst, Benjamin A.; Beecher, Sierra D.; Dodgen, Adam C.; Jensen, Kaare Hartvig; Holbrook, N. Michele Published in: eLife Link to article, DOI: 10.7554/eLife.15341 Publication date: 2016 Document Version Publisher's PDF, also known as Version of record Link back to DTU Orbit Citation (APA): Knoblauch, M., Knoblauch, J., Mullendore, D. L., Savage, J. A., Babst, B. A., Beecher, S. D., Dodgen, A. C., Jensen, K. H., & Holbrook, N. M. (2016). Testing the Münch hypothesis of long distance phloem transport in plants. eLife, 5. https://doi.org/10.7554/eLife.15341 General rights Copyright and moral rights for the publications made accessible in the public portal are retained by the authors and/or other copyright owners and it is a condition of accessing publications that users recognise and abide by the legal requirements associated with these rights. Users may download and print one copy of any publication from the public portal for the purpose of private study or research. You may not further distribute the material or use it for any profit-making activity or commercial gain You may freely distribute the URL identifying the publication in the public portal If you believe that this document breaches copyright please contact us providing details, and we will remove access to the work immediately and investigate your claim. RESEARCH ARTICLE Testing -
Redalyc.Stem and Root Anatomy of Two Species of Echinopsis
Revista Mexicana de Biodiversidad ISSN: 1870-3453 [email protected] Universidad Nacional Autónoma de México México dos Santos Garcia, Joelma; Scremin-Dias, Edna; Soffiatti, Patricia Stem and root anatomy of two species of Echinopsis (Trichocereeae: Cactaceae) Revista Mexicana de Biodiversidad, vol. 83, núm. 4, diciembre, 2012, pp. 1036-1044 Universidad Nacional Autónoma de México Distrito Federal, México Available in: http://www.redalyc.org/articulo.oa?id=42525092001 How to cite Complete issue Scientific Information System More information about this article Network of Scientific Journals from Latin America, the Caribbean, Spain and Portugal Journal's homepage in redalyc.org Non-profit academic project, developed under the open access initiative Revista Mexicana de Biodiversidad 83: 1036-1044, 2012 DOI: 10.7550/rmb.28124 Stem and root anatomy of two species of Echinopsis (Trichocereeae: Cactaceae) Anatomía de la raíz y del tallo de dos especies de Echinopsis (Trichocereeae: Cactaceae) Joelma dos Santos Garcia1, Edna Scremin-Dias1 and Patricia Soffiatti2 1Universidade Federal de Mato Grosso do Sul, CCBS, Departamento de Biologia, Programa de Pós Graduação em Biologia Vegetal Cidade Universitária, S/N, Caixa Postal 549, CEP 79.070.900 Campo Grande, MS, Brasil. 2Universidade Federal do Paraná, SCB, Departamento de Botânica, Programa de Pós-Graduação em Botânica, Caixa Postal 19031, CEP 81.531.990 Curitiba, PR, Brasil. [email protected] Abstract. This study characterizes and compares the stem and root anatomy of Echinopsis calochlora and E. rhodotricha (Cactaceae) occurring in the Central-Western Region of Brazil, in Mato Grosso do Sul State. Three individuals of each species were collected, fixed, stored and prepared following usual anatomy techniques, for subsequent observation in light and scanning electronic microscopy. -
Tree Anatomy Stems and Branches
Tree Anatomy Series WSFNR14-13 Nov. 2014 COMPONENTSCOMPONENTS OFOF PERIDERMPERIDERM by Dr. Kim D. Coder, Professor of Tree Biology & Health Care Warnell School of Forestry & Natural Resources, University of Georgia Around tree roots, stems and branches is a complex tissue. This exterior tissue is the environmental face of a tree open to all sorts of site vulgarities. This most exterior of tissue provides trees with a measure of protection from a dry, oxidative, heat and cold extreme, sunlight drenched, injury ridden site. The exterior of a tree is both an ecological super highway and battle ground – comfort and terror. This exterior is unique in its attributes, development, and regeneration. Generically, this tissue surrounding a tree stem, branch and root is loosely called bark. The tissues of a tree, outside or more exterior to the xylem-containing core, are varied and complexly interwoven in a relatively small space. People tend to see and appreciate the volume and physical structure of tree wood and dismiss the remainder of stem, branch and root. In reality, tree life is focused within these more exterior thin tissue sets. Outside of the cambium are tissues which include transport cells, structural support cells, generation cells, and cells positioned to help, protect, and sustain other cells. All of this life is smeared over the circumference of a predominately dead physical structure. Outer Skin Periderm (jargon and antiquated term = bark) is the most external of tree tissues providing protection, water conservation, insulation, and environmental sensing. Periderm is a protective tissue generated over and beyond live conducting and non-conducting cells of the food transport system (phloem). -
Methods for Measuring Frost Tolerance of Conifers: a Systematic Map
Review Methods for Measuring Frost Tolerance of Conifers: A Systematic Map Anastasia-Ainhoa Atucha Zamkova *, Katherine A. Steele and Andrew R. Smith School of Natural Sciences, Bangor University, Bangor LL57 2UW, Gwynedd, UK; [email protected] (K.A.S.); [email protected] (A.R.S.) * Correspondence: [email protected] Abstract: Frost tolerance is the ability of plants to withstand freezing temperatures without unrecov- erable damage. Measuring frost tolerance involves various steps, each of which will vary depending on the objectives of the study. This systematic map takes an overall view of the literature that uses frost tolerance measuring techniques in gymnosperms, focusing mainly on conifers. Many different techniques have been used for testing, and there has been little change in methodology since 2000. The gold standard remains the field observation study, which, due to its cost, is frequently substituted by other techniques. Closed enclosure freezing tests (all non-field freezing tests) are done using various types of equipment for inducing artificial freezing. An examination of the literature indicates that several factors have to be controlled in order to measure frost tolerance in a manner similar to observation in a field study. Equipment that allows controlling the freezing rate, frost exposure time and thawing rate would obtain results closer to field studies. Other important factors in study design are the number of test temperatures used, the range of temperatures selected and the decrements between the temperatures, which should be selected based on expected frost tolerance of the tissue and species. Citation: Atucha Zamkova, A.-A.; Steele, K.A.; Smith, A.R. -
Dicot/Monocot Root Anatomy the Figure Shown Below Is a Cross Section of the Herbaceous Dicot Root Ranunculus. the Vascular Tissu
Dicot/Monocot Root Anatomy The figure shown below is a cross section of the herbaceous dicot root Ranunculus. The vascular tissue is in the very center of the root. The ground tissue surrounding the vascular cylinder is the cortex. An epidermis surrounds the entire root. The central region of vascular tissue is termed the vascular cylinder. Note that the innermost layer of the cortex is stained red. This layer is the endodermis. The endodermis was derived from the ground meristem and is properly part of the cortex. All the tissues inside the endodermis were derived from procambium. Xylem fills the very middle of the vascular cylinder and its boundary is marked by ridges and valleys. The valleys are filled with phloem, and there are as many strands of phloem as there are ridges of the xylem. Note that each phloem strand has one enormous sieve tube member. Outside of this cylinder of xylem and phloem, located immediately below the endodermis, is a region of cells called the pericycle. These cells give rise to lateral roots and are also important in secondary growth. Label the tissue layers in the following figure of the cross section of a mature Ranunculus root below. 1 The figure shown below is that of the monocot Zea mays (corn). Note the differences between this and the dicot root shown above. 2 Note the sclerenchymized endodermis and epidermis. In some monocot roots the hypodermis (exodermis) is also heavily sclerenchymized. There are numerous xylem points rather than the 3-5 (occasionally up to 7) generally found in the dicot root. -
Anatomical Traits Related to Stress in High Density Populations of Typha Angustifolia L
http://dx.doi.org/10.1590/1519-6984.09715 Original Article Anatomical traits related to stress in high density populations of Typha angustifolia L. (Typhaceae) F. F. Corrêaa*, M. P. Pereiraa, R. H. Madailb, B. R. Santosc, S. Barbosac, E. M. Castroa and F. J. Pereiraa aPrograma de Pós-graduação em Botânica Aplicada, Departamento de Biologia, Universidade Federal de Lavras – UFLA, Campus Universitário, CEP 37200-000, Lavras, MG, Brazil bInstituto Federal de Educação, Ciência e Tecnologia do Sul de Minas Gerais – IFSULDEMINAS, Campus Poços de Caldas, Avenida Dirce Pereira Rosa, 300, CEP 37713-100, Poços de Caldas, MG, Brazil cInstituto de Ciências da Natureza, Universidade Federal de Alfenas – UNIFAL, Rua Gabriel Monteiro da Silva, 700, CEP 37130-000, Alfenas, MG, Brazil *e-mail: [email protected] Received: June 26, 2015 – Accepted: November 9, 2015 – Distributed: February 28, 2017 (With 3 figures) Abstract Some macrophytes species show a high growth potential, colonizing large areas on aquatic environments. Cattail (Typha angustifolia L.) uncontrolled growth causes several problems to human activities and local biodiversity, but this also may lead to competition and further problems for this species itself. Thus, the objective of this study was to investigate anatomical modifications on T. angustifolia plants from different population densities, once it can help to understand its biology. Roots and leaves were collected from natural populations growing under high and low densities. These plant materials were fixed and submitted to usual plant microtechnique procedures. Slides were observed and photographed under light microscopy and images were analyzed in the UTHSCSA-Imagetool software. The experimental design was completely randomized with two treatments and ten replicates, data were submitted to one-way ANOVA and Scott-Knott test at p<0.05. -
Eudicots Monocots Stems Embryos Roots Leaf Venation Pollen Flowers
Monocots Eudicots Embryos One cotyledon Two cotyledons Leaf venation Veins Veins usually parallel usually netlike Stems Vascular tissue Vascular tissue scattered usually arranged in ring Roots Root system usually Taproot (main root) fibrous (no main root) usually present Pollen Pollen grain with Pollen grain with one opening three openings Flowers Floral organs usually Floral organs usually in in multiples of three multiples of four or five © 2014 Pearson Education, Inc. 1 Reproductive shoot (flower) Apical bud Node Internode Apical bud Shoot Vegetative shoot system Blade Leaf Petiole Axillary bud Stem Taproot Lateral Root (branch) system roots © 2014 Pearson Education, Inc. 2 © 2014 Pearson Education, Inc. 3 Storage roots Pneumatophores “Strangling” aerial roots © 2014 Pearson Education, Inc. 4 Stolon Rhizome Root Rhizomes Stolons Tubers © 2014 Pearson Education, Inc. 5 Spines Tendrils Storage leaves Stem Reproductive leaves Storage leaves © 2014 Pearson Education, Inc. 6 Dermal tissue Ground tissue Vascular tissue © 2014 Pearson Education, Inc. 7 Parenchyma cells with chloroplasts (in Elodea leaf) 60 µm (LM) © 2014 Pearson Education, Inc. 8 Collenchyma cells (in Helianthus stem) (LM) 5 µm © 2014 Pearson Education, Inc. 9 5 µm Sclereid cells (in pear) (LM) 25 µm Cell wall Fiber cells (cross section from ash tree) (LM) © 2014 Pearson Education, Inc. 10 Vessel Tracheids 100 µm Pits Tracheids and vessels (colorized SEM) Perforation plate Vessel element Vessel elements, with perforated end walls Tracheids © 2014 Pearson Education, Inc. 11 Sieve-tube elements: 3 µm longitudinal view (LM) Sieve plate Sieve-tube element (left) and companion cell: Companion cross section (TEM) cells Sieve-tube elements Plasmodesma Sieve plate 30 µm Nucleus of companion cell 15 µm Sieve-tube elements: longitudinal view Sieve plate with pores (LM) © 2014 Pearson Education, Inc.