Nucleic Acids Research
Total Page:16
File Type:pdf, Size:1020Kb
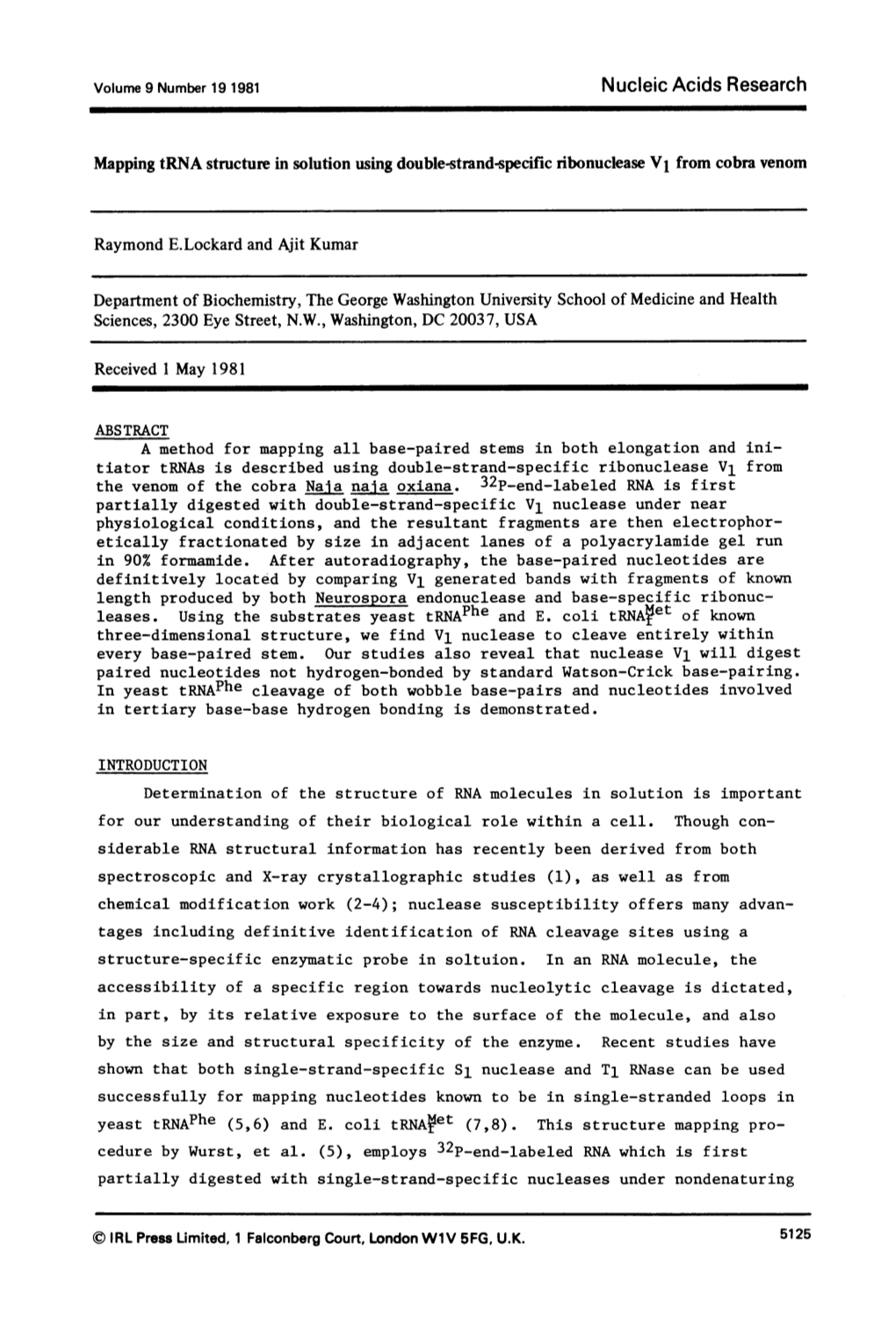
Load more
Recommended publications
-
Riboswitch-Targeted Drug Discovery: Investigation of Factors That Affect the T Box
Riboswitch-targeted Drug Discovery: Investigation of Factors that Affect the T Box Transcription Antitermination Mechanism A dissertation presented to the faculty of the College of Arts and Sciences of Ohio University In partial fulfillment of the requirements for the degree Doctor of Philosophy Chunxi Zeng April 2016 © 2016 Chunxi Zeng. All Rights Reserved. 2 This dissertation titled Riboswitch-targeted Drug Discovery: Investigation of Factors that Affect the T Box Transcription Antitermination Mechanism by CHUNXI ZENG has been approved for the Department of Chemistry and Biochemistry and the College of Arts and Sciences by Jennifer V. Hines Professor of Chemistry and Biochemistry Robert Frank Dean, College of Arts and Sciences 3 ABSTRACT ZENG, CHUNXI, Ph.D., April 2016, Molecular and Cellular Biology Riboswitch-targeted Drug Discovery: Investigation of Factors that Affect the T Box Transcription Antitermination Mechanism Director of Dissertation: Jennifer V. Hines The T box riboswitch is a regulation mechanism at the level of transcription or translation which controls expression of amino acids related genes, including a lot of essential genes, in many bacteria. The T box riboswitch interacts with cognate tRNAs and senses their aminoacylation status. A charged cognate tRNA allows formation of the thermodynamically more stable terminator structure which induces transcription termination. An uncharged tRNA stabilizes the alternative antiterminator structure and prevents formation of the terminator. Transcription proceeds and leads to expression of the downstream gene(s). The T box riboswitch is a novel and promising drug target since multiple genes essential to bacterial survival are regulated by this mechanism in many pathogenic bacteria. In order to further study the T box riboswitch mechanism and screen a synthetic ligands library, a fluorescently monitored multi-round in vitro antitermination assay with an enhanced throughput was successfully developed and comprehensively evaluated. -
Ribosomal Protein S1 Induces a Conformational Change of Tmrna; More Than One Protein S1 Per Molecule of Tmrna
Ribosomal protein S1 induces a conformational change of tmRNA; more than one protein S1 per molecule of tmRNA. Val´erieBordeau, Brice Felden To cite this version: Val´erieBordeau, Brice Felden. Ribosomal protein S1 induces a conformational change of tm- RNA; more than one protein S1 per molecule of tmRNA.. Biochimie, Elsevier, 2002, 84 (8), pp.723-9. <inserm-00718154> HAL Id: inserm-00718154 http://www.hal.inserm.fr/inserm-00718154 Submitted on 16 Jul 2012 HAL is a multi-disciplinary open access L'archive ouverte pluridisciplinaire HAL, est archive for the deposit and dissemination of sci- destin´eeau d´ep^otet `ala diffusion de documents entific research documents, whether they are pub- scientifiques de niveau recherche, publi´esou non, lished or not. The documents may come from ´emanant des ´etablissements d'enseignement et de teaching and research institutions in France or recherche fran¸caisou ´etrangers,des laboratoires abroad, or from public or private research centers. publics ou priv´es. Biochimie 84 (2002) 723–729 Ribosomal protein S1 induces a conformational change of tmRNA; more than one protein S1 per molecule of tmRNA Valérie Bordeau, Brice Felden * Laboratoire de Biochimie Pharmaceutique, Faculté de Pharmacie, Université de Rennes I, UPRES Jeune Equipe 2311, 2, avenue du Pr. Léon-Bernard, 35043 Rennes cedex, France Received 15 March 2002; accepted 23 April 2002 Abstract tmRNA (10Sa RNA, ssrA) acts to rescue stalled bacterial ribosomes while encoding a peptide tag added trans-translationally to the nascent peptide, targeting it for proteolysis. Ribosomal protein S1 is required for tmRNA binding to isolated and poly U-programmed ribosomes. -
Recognizes Transfer-Messenger RNA with Specificity; a Functional Complex Prior to Entering the Ribosome? Reynald Gillet, Brice Felden
Transfer RNA(Ala) recognizes transfer-messenger RNA with specificity; a functional complex prior to entering the ribosome? Reynald Gillet, Brice Felden To cite this version: Reynald Gillet, Brice Felden. Transfer RNA(Ala) recognizes transfer-messenger RNA with specificity; a functional complex prior to entering the ribosome?. EMBO Journal, EMBO Press, 2001, 20 (11), pp.2966-76. 10.1093/emboj/20.11.2966. inserm-00718204 HAL Id: inserm-00718204 https://www.hal.inserm.fr/inserm-00718204 Submitted on 16 Jul 2012 HAL is a multi-disciplinary open access L’archive ouverte pluridisciplinaire HAL, est archive for the deposit and dissemination of sci- destinée au dépôt et à la diffusion de documents entific research documents, whether they are pub- scientifiques de niveau recherche, publiés ou non, lished or not. The documents may come from émanant des établissements d’enseignement et de teaching and research institutions in France or recherche français ou étrangers, des laboratoires abroad, or from public or private research centers. publics ou privés. The EMBO Journal Vol. 20 No. 11 pp. 2966±2976, 2001 Transfer RNAAla recognizes transfer-messenger RNA with speci®city; a functional complex prior to entering the ribosome? Reynald Gillet and Brice Felden1 involved in cellular adaptation to lactose availability (Abo et al., 2000). tmRNA function is also required for the Laboratoire de Biochimie Pharmaceutique, Faculte de Pharmacie, Universite de Rennes I, UPRES Jeune Equipe 2311, IFR 97, ef®cient growth of Bacillus subtilis under various stresses 2 avenue du Pr LeÂon Bernard, 35043 Rennes, France (Muto et al., 2000). Altogether, evidence suggests that tmRNA expression becomes crucial when bacteria have to 1Corresponding author e-mail: [email protected] adapt to environmental changes. -
Open Thesis Kayceeaquarles.Pdf
The Pennsylvania State University The Graduate School Eberly College of Science THE IMPACT OF PRIMARY MICRORNA STRUCTURE ON RECOGNITION BY THE MICROPROCESSOR COMPLEX IN MICRORNA MATURATION A Dissertation in Chemistry by Kaycee Andrea Quarles © 2015 Kaycee Andrea Quarles Submitted in Partial Fulfillment of the Requirements for the Degree of Doctor of Philosophy May 2015 ii The dissertation of Kaycee Andrea Quarles was reviewed and approved* by the following: Scott A. Showalter Associate Professor of Chemistry Dissertation Advisor Chair of Committee Philip C. Bevilacqua Professor of Chemistry Christine D. Keating Professor of Chemistry Katsuhiko Murakami Associate Professor of Biochemistry and Molecular Biology Barbara J. Garrison Shapiro Professor of Chemistry Head of the Department of Chemistry *Signatures are on file in the Graduate School. iii ABSTRACT Since their discovery over a decade ago, thousands of microRNAs (miRNAs) have been found across all multicellular organisms. These RNAs in combination with small interfering RNAs (siRNAs) make up the RNA silencing pathway, also called the RNA interference (RNAi) pathway. Mature miRNAs are ~22-nucleotide-long, single-stranded non-coding RNAs that participate in various cellular, developmental, and differentiation processes via post- transcriptional regulation of gene expression for more than 90% of human genes. Therefore, these key RNAs have been linked to several disease states including cancer, neurodegenerative diseases, cardiac disease, diabetes, and numerous viral diseases. Recently, they have become a key target for the medical RNA therapeutics community. The human canonical miRNA maturation pathway involves a series of cleavage steps beginning in the nucleus and ending in the cytoplasm, where the final mature miRNA down- regulates gene expression via “silencing” messenger RNA translation. -
Open Heinicke 04 26 10 Thesis
The Pennsylvania State University The Graduate School Department of Chemistry REGULATION OF THE PROTEIN KINASE PKR BY HIGHER-ORDER RNA SECONDARY AND TERTIARY STRUCTURES A Dissertation in Chemistry by Laurie A. Heinicke © 2010 Laurie A. Heinicke Submitted in Partial Fulfillment of the Requirements for the Degree of Doctor of Philosophy August 2010 ii The dissertation of Laurie A. Heinicke was reviewed and approved* by the following Philip C. Bevilacqua Professor of Chemistry Dissertation Advisor Chair of Committee Christine D. Keating Associate Professor of Chemistry Scott Showalter Assistant Professor of Chemistry Andrey Krasilnikov Assistant Professor of Biochemistry and Molecular Biology Barbara Garrison Professor of Chemistry Head of the Department of Chemistry *Signatures are on file in the Graduate School iii ABSTRACT A single strand of RNA can fold into multiple structures, often forming complex secondary and tertiary stuctures. Many factors influence RNA folding, including proteins, small molecules, salt, temperature, and pH. Human protein kinase PKR is a component of the innate immune response, and is activated by long stretches of double- stranded RNA (dsRNA), but is inhibited by highly structured or short dsRNAs. Activation of PKR often results in inhibition of protein synthesis. The types of RNAs encountered by PKR in the cell are diverse in structure and function, including mRNA, miRNA, tRNA, rRNA and viral RNAs. While PKR regulation by human cellular RNAs has not been extensively studied, it is known that PKR is activated by viral dsRNA genomes and long dsRNA replicative intermediates. The work presented in this thesis focused primarily on trapping and characterizing a unique RNA fold and then examining PKR regulation by that RNA. -
Structure, Function and Evolution of Armless Mitochondrial Trnas Tina Jühling
Structure, function and evolution of armless mitochondrial tRNAs Tina Jühling To cite this version: Tina Jühling. Structure, function and evolution of armless mitochondrial tRNAs. Parasitology. Uni- versité de Strasbourg; Karl-Marx-Universität Leipzig, 2016. English. NNT : 2016STRAJ119. tel- 01673831v2 HAL Id: tel-01673831 https://tel.archives-ouvertes.fr/tel-01673831v2 Submitted on 11 Jan 2018 HAL is a multi-disciplinary open access L’archive ouverte pluridisciplinaire HAL, est archive for the deposit and dissemination of sci- destinée au dépôt et à la diffusion de documents entific research documents, whether they are pub- scientifiques de niveau recherche, publiés ou non, lished or not. The documents may come from émanant des établissements d’enseignement et de teaching and research institutions in France or recherche français ou étrangers, des laboratoires abroad, or from public or private research centers. publics ou privés. UNIVERSITÉ DE STRASBOURG ÉCOLE DOCTORALE DES SCIENCES DE LA VIE ET DE LA SANTÉ Architecture et Réactivité de l’ARN – UPR 9002 du CNRS et / und UNIVERSITÄT LEIPZIG FAKULTÄT FÜR BIOWISSENSCHAFTEN, PHARMAZIE UND PSYCHOLOGIE THÈSE / DISSERTATION présentée par / vorgelegt von Tina MÜLLER (ép. JÜHLING) soutenue le / verteidigt am 14.12.2016 pour obtenir le grade de Docteur de l’université de Strasbourg (Dr.) zur Erlangung des akademischen Grades Doctor rerum naturalium (Dr. rer. nat.) Discipline/Spécialité : Aspects moléculaires et cellulaires de la biologie im Fachgebiet : Biochemie ARNt « manchots » structure, fonctionnalité et évolution THÈSE co-dirigée par: Prof. FLORENTZ Catherine Professeur, IBMC, Université de Strasbourg Prof. MÖRL Mario Professeur, Institut de Biochimie, Université de Leipzig RAPPORTEURS : Prof. LAYER Gunhild Professeur, Institut de Biochimie, Université de Leipzig Prof. -
Ribosomal Movement Impeded at a Pseudoknot Required for Frameshifting
Proc. Nati. Acad. Sci. USA Vol. 89, pp. 8636-8640, September 1992 Biochemistry Ribosomal movement impeded at a pseudoknot required for frameshifting (doubkl-stded RNA vlrus/Saccharomyces cerevisiae vfrus/trnsaation/yeast) CHIALING Tu, TZY-HWA TZENG, AND JEREMY A. BRUENN* Department of Biological Sciences, State University of New York, Buffalo, NY 14260 Communicated by Edwin D. Kilbourne, June 15, 1992 (receivedfor review April 7, 1992) ABSTRACT Translational frameshifting sometimes occurs backward one base to erroneously read the Gly-Phe codons when ribosomes encounter a "shift" site preceding a region of (T.-H.T., unpublished data). unusual secondary strucure, which in at least three cases is In this work, ribosomes are demonstrated to progress known to be a pseudoknot. We provide evidence that ribosones slowly through the pseudoknot, so that "paused" ribosomes have a decreased rate of movement through a pseudoknot accumulate, apparently flush up against its first stem. Mu- required for fram ing. These paused ribosomes are directly tations that eliminate either stem of the pseudoknot greatly situated over the shift sequence. Ribosomal pausing appears to reduce ribosomal frameshifting and ribosomal pausing at this be necessary but not sufficent for frameshfting. site. Only the secondary structure ofthe RNA is important in this region, not its primary sequence. Ribosomal frameshifting is the shifting ofribosomes from one MATERIALS AND METHODS a mRNA reading frame to another at a specific sequence on Vectors for Detection of FrameshUtng. The pGEM7ZCTU during translation. There are numerous examples ofribosomal family ofvectors for detection of ScV frameshifting has been frameshifting in prokaryotic and eukaryotic systems (1, 2). -
Dependent Precursors of 24 Nt Sirnas Guiding De Novo DNA Methylation
RESEARCH ARTICLE Identification of Pol IV and RDR2- dependent precursors of 24 nt siRNAs guiding de novo DNA methylation in Arabidopsis Todd Blevins1,2,3†‡, Ram Podicheti4,5†, Vibhor Mishra2,3, Michelle Marasco2,3, Jing Wang2,3, Doug Rusch4, Haixu Tang5, Craig S Pikaard1,2,3* 1Howard Hughes Medical Institute, Indiana University, Bloomington, United States; 2Department of Biology, Indiana University, Bloomington, United States; 3Department of Molecular and Cellular Biochemistry, Indiana University, Bloomington, United States; 4Center for Genomics and Bioinformatics, Indiana University, Bloomington, United States; 5School of Informatics and Computing, Indiana University, Bloomington, United States Abstract In Arabidopsis thaliana, abundant 24 nucleotide small interfering RNAs (24 nt siRNA) *For correspondence: cpikaard@ guide the cytosine methylation and silencing of transposons and a subset of genes. 24 nt siRNA indiana.edu biogenesis requires nuclear RNA polymerase IV (Pol IV), RNA-dependent RNA polymerase 2 (RDR2) †These authors contributed and DICER-like 3 (DCL3). However, siRNA precursors are mostly undefined. We identified Pol IV equally to this work and RDR2-dependent RNAs (P4R2 RNAs) that accumulate in dcl3 mutants and are diced into 24 nt Present address: ‡Institut de RNAs by DCL3 in vitro. P4R2 RNAs are mostly 26-45 nt and initiate with a purine adjacent to a Biologie Mole´culaire des Plantes pyrimidine, characteristics shared by Pol IV transcripts generated in vitro. RDR2 terminal du Centre national de la transferase activity, also demonstrated in vitro, may account for occasional non-templated recherche scientifique, Universite´ nucleotides at P4R2 RNA 3’ termini. The 24 nt siRNAs primarily correspond to the 5’ or 3’ ends of de Strasbourg, Strasbourg, P4R2 RNAs, suggesting a model whereby siRNAs are generated from either end of P4R2 duplexes France by single dicing events. -
RNA Molecules Stimulate Prion Protein Conversion
letters to nature between 0.2 and 1.0 in nestlings. As not all individuals were typed with the same set of pedigrees and (2) distinguishing full-sib pair members. Anim. Genet. 25, 37–44 (1994). microsatellite markers, we calculated standardized individual heterozygosity10 29. Pemberton, J. M., Coltman, D. W., Coulson, T. N. & Slate, J. in Microsatellites, Evolution and (proportion of heterozygous loci/mean heterozygosity of typed loci). This measure is used Applications (eds Goldstein, D. B. & Schlo¨tterer, C.) 151–164 (Oxford Univ. Press, Oxford, 1999). in all analyses and is referred to as ‘individual heterozygosity’ for simplicity. We also 30. Turelli, M. & Ginzburg, L. R. Should individual fitness increase with heterozygosity? Genetics 104, calculated two other, previously suggested measures of individual genetic diversity: 191–209 (1983). internal relatedness10 and mean d2-value29 where d 2 is the squared length difference between the alleles at a locus. Heterozygosity, standardized heterozygosity and internal Acknowledgements We thank D. Blomqvist, S. Griffith, D. Hasselquist, L. Keller, M. Milinski, relatedness were highly correlated (all r . 0.89, P , 0.0001) and all three measures led to A. Peters, B. Sheldon, C. Wedekind and D. Zeh for comments on the manuscript; K. Carter, similar conclusions (data not shown). Mean d 2-values were weakly correlated with the D. Kaulfuss, H. Kunc, K. Peer, A. Po¨sel and A. Tu¨rk for help with field and laboratory work; other measures (r ¼ 0.24–0.32, all P , 0.0001) and explained comparatively little S. Andersson for computing the colour variables; and H. Winkler (Konrad Lorenz Institute for variation in individual fitness. -
Pathology-Related Mutation A7526G (A9G) Helps in the Understanding of the 3D Structural Core of Human Mitochondrial Trnaasp
Downloaded from rnajournal.cshlp.org on September 27, 2021 - Published by Cold Spring Harbor Laboratory Press LETTER TO THE EDITOR Pathology-related mutation A7526G (A9G) helps in the understanding of the 3D structural core of human mitochondrial tRNAAsp MARIE MESSMER, AGNE`S GAUDRY, MARIE SISSLER, and CATHERINE FLORENTZ Architecture et Re´activite´ de l’ARN, Institut de Biologie Mole´culaire et Cellulaire, Centre National de la Recherche Scientifique, Universite´ de Strasbourg, 67084 Strasbourg, France ABSTRACT More than 130 mutations in human mitochondrial tRNA (mt-tRNA) genes have been correlated with a variety of neurodegenerative and neuromuscular disorders. Their molecular impacts are of mosaic type, affecting various stages of tRNA biogenesis, structure, and/or functions in mt-translation. Knowledge of mammalian mt-tRNA structures per se remains scarce however. Primary and secondary structures deviate from classical tRNAs, while rules for three-dimensional (3D) folding are almost unknown. Here, we take advantage of a myopathy-related mutation A7526G (A9G) in mt-tRNAAsp to investigate both the primary molecular impact underlying the pathology and the role of nucleotide 9 in the network of 3D tertiary interactions. Experimental evidence is presented for existence of a 9-12-23 triple in human mt-tRNAAsp with a strongly conserved interaction scheme in mammalian mt-tRNAs. Mutation A7526G disrupts the triple interaction and in turn reduces aspartylation efficiency. Keywords: mammalian mitochondria; tRNA; structure probing in solution; pathology-related mutations; mitochondrial disorder INTRODUCTION (e.g., Helm et al. 2000), pathologic or polymorphic inci- dence of a mutation can still not be predicted (Florentz and The human mitochondrial (mt) genome codes for 13 pro- Sissler 2001; Florentz et al. -
Inhibitors of Protein-RNA Complexation That
14204 Biochemistry 1998, 37, 14204-14212 Inhibitors of Protein-RNA Complexation That Target the RNA: Specific Recognition of Human Immunodeficiency Virus Type 1 TAR RNA by Small Organic Molecules Houng-Yau Mei,*,‡ Mei Cui,‡ Andrea Heldsinger,§ Shannon M. Lemrow,‡ Joseph A. Loo,‡ Kristin A. Sannes-Lowery,‡ Lamia Sharmeen,§ and Anthony W. Czarnik‡,| Department of Chemistry and Department of Therapeutics, Parke-DaVis Pharmaceutical Research, DiVision of Warner-Lambert Company, 2800 Plymouth Road, Ann Arbor, Michigan 48106 ReceiVed June 17, 1998; ReVised Manuscript ReceiVed August 12, 1998 ABSTRACT: TAR RNA represents an attractive target for the intervention of human immunodeficiency virus type 1 (HIV-1) replication by small molecules. We now describe three small molecule inhibitors of the HIV-1 Tat-TAR interaction that target the RNA, not the protein. The chemical structures and RNA binding characteristics of these inhibitors are unique for each molecule. Results from various biochemical and spectroscopic methods reveal that each of the three Tat-TAR inhibitors recognizes a different structural feature at the bulge, lower stem, or loop region of TAR. Furthermore, one of these Tat-TAR inhibitors has been demonstrated, in cellular environments, to inhibit (a) a TAR-dependent, Tat-activated transcription and (b) the replication of HIV-1 in a latently infectious model. Drug discovery has traditionally involved a search for substrates for self-splicing reactions (6, 7). Can such inhibitors of protein complexation to small molecules (e.g., structured nucleic acids display sufficient shape diversity to substrates or ligands) or macromolecules (e.g., other proteins, permit the complexation of a small molecule at one site in nucleic acids, or polysaccharides). -
The Trna-Like Domains of E Coli and A.Aeolicus Transfer-Messenger RNA: Structural and Functional Studies
The tRNA-like domains of E coli and A.aeolicus transfer-messenger RNA: structural and functional studies. Cyril Gaudin, Sylvie Nonin-Lecomte, Carine Tisné, Sophie Corvaisier, Valérie Bordeau, Frédéric Dardel, Brice Felden To cite this version: Cyril Gaudin, Sylvie Nonin-Lecomte, Carine Tisné, Sophie Corvaisier, Valérie Bordeau, et al.. The tRNA-like domains of E coli and A.aeolicus transfer-messenger RNA: structural and functional stud- ies.. Journal of Molecular Biology, Elsevier, 2003, 331 (2), pp.457-71. 10.1016/S0022-2836(03)00760- 5. hal-00219759 HAL Id: hal-00219759 https://hal.archives-ouvertes.fr/hal-00219759 Submitted on 27 Jan 2008 HAL is a multi-disciplinary open access L’archive ouverte pluridisciplinaire HAL, est archive for the deposit and dissemination of sci- destinée au dépôt et à la diffusion de documents entific research documents, whether they are pub- scientifiques de niveau recherche, publiés ou non, lished or not. The documents may come from émanant des établissements d’enseignement et de teaching and research institutions in France or recherche français ou étrangers, des laboratoires abroad, or from public or private research centers. publics ou privés. The tRNA-like domain of tmRNA The tRNA-like domains of E. coli and A. aeolicus Transfer-messenger RNA: Structural and Functional studies. Cyril Gaudin1+, Sylvie Nonin-Lecomte2+, Carine Tisné2, Sophie Corvaisier1, Valérie Bordeau1, Frédéric Dardel2* and Brice Felden1. 1Laboratoire de Biochimie Pharmaceutique UPRES JE2311, Faculté de Pharmacie, Université de Rennes I, 2 avenue du Pr. Léon Bernard, 35043 Rennes, France. 2Laboratoire de Cristallographie et RMN Biologiques, UMR8015 CNRS, Faculté de Pharmacie, Université Paris 5, 4, avenue de l’Observatoire, 75006, Paris, France.