Proceedings of the Workshop on the Science of Atmospheric Trace Gases, 2004
Total Page:16
File Type:pdf, Size:1020Kb
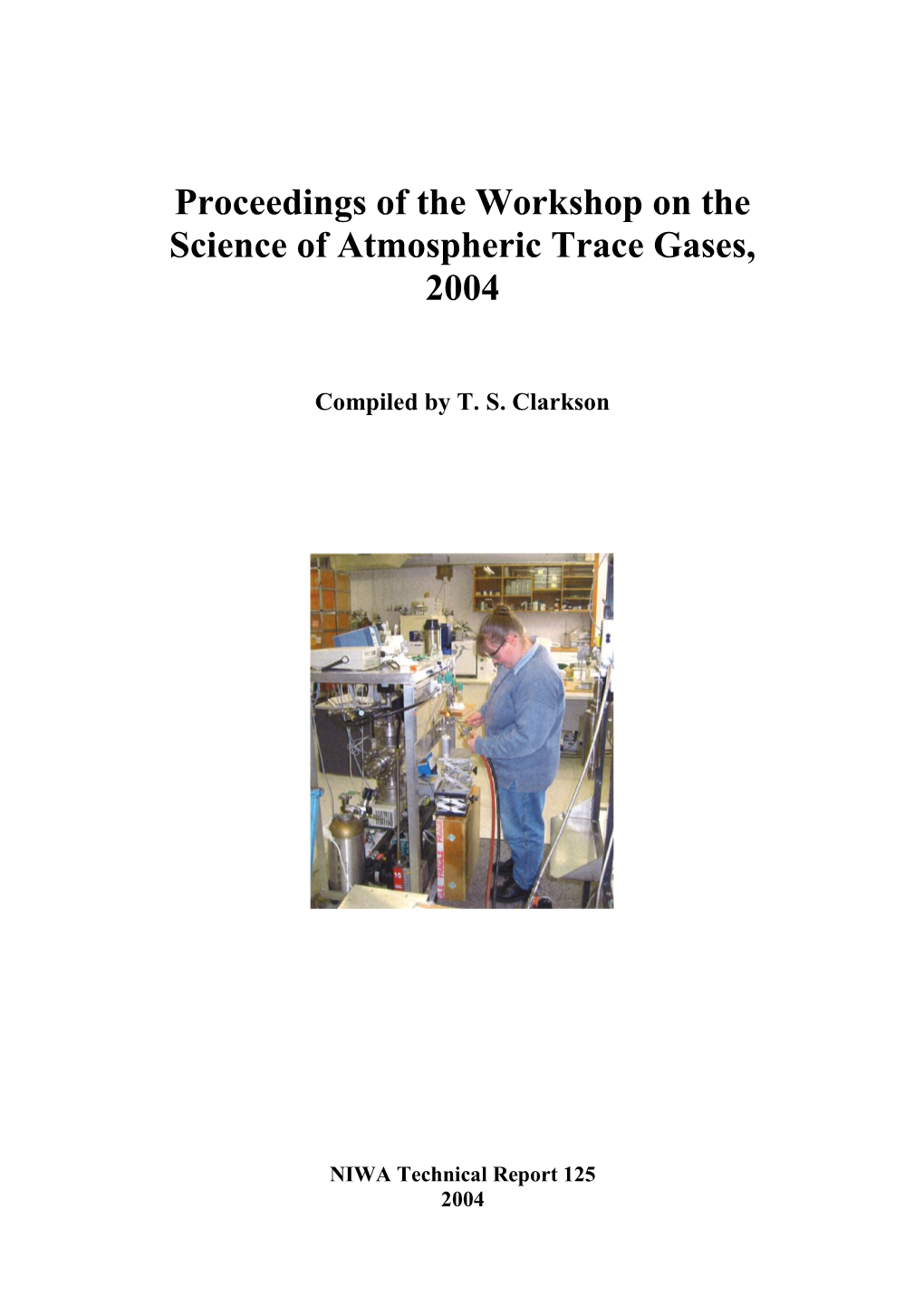
Load more
Recommended publications
-
Water& Atmosphere
Water & Atmosphere March 2012 Healing waters Cleaning up the Rotorua lakes Unsightly growth? Mangroves on the march Pig power It's a gas David Wratt Beyond belief Invaders from inner space Checking for stowaways Water & Atmosphere March 2012 Cover A fly fisherman tries his luck on a still Rotorua morning. Many of the district’s lakes are polluted by nitrogen and phosphorus runoff, and five are the focus of a multi-agency cleanup programme. Returning those lakes to health is seen by some as the foundation of the city’s economic future. (David Hallett/Hedgehog House) Water & Atmosphere is published by the National Institute of Water & Atmospheric Research (NIWA). It is available online at www.niwa.co.nz/pubs/wa Enquiries to: The Editor 4 Water & Atmosphere NIWA Editorial Private Bag 14901 Kilbirnie Our environment offers one of the best Wellington 6241 investment portfolios, says John Morgan New Zealand email: [email protected] ©National Institute of Water & Atmospheric Research Ltd 2012 ISSN 1172-1014 5 Water & Atmosphere team: In brief Editor: Dave Hansford Cold case, ebbs and flows, stalking sound, Production: NIWA Communications and Marketing team COkudos, our so-so summer 28 Portfolio More great shots from the coalface by NIWA staff 38 Q&A Elementary, my dear Watson: clues from Water & Atmosphere is produced using vegetable-based inks on stable isotopes paper made from FSC certifed mixed-source fibres under the ISO 14001 environmental management system. 2 Water & Atmosphere March 2012 www.niwa.co.nz 16 Healing waters P soup at Rotorua 11 Mangroves on the march Thickets, and fast 24 Pig power Gas works 32 36 Invaders from David Wratt inner space NIWA's climate chief on science, steadfastness Looking for trouble and spark plugs www.niwa.co.nz Water & Atmosphere March 2012 3 Editorial Realising our assets In November 1994, the skipper of a Russian super trawler, the Yefim Gorbenko, put his vessel into Calliope dry dock in Devonport, complaining of excessive fuel consumption and high engine temperatures. -
A Summary of the Assessment Process of the Intergovernmental Panel on Climate Change
A Summary of the Assessment Process of the Intergovernmental Panel on Climate Change An invited presentation to the New Zealand Emissions Trading Scheme Review Committee Martin Manning Andy Reisinger David Wratt New Zealand Climate Change Research Institute, Victoria University of Wellington February 2009 New Zealand Climate Change Research Institute School of Government Victoria University of Wellington PO Box 600 Wellington New Zealand Report No: NZCCRI 2009-01 The Process of Climate Change Science Assessment as Carried Out by the Intergovernmental Panel on Climate Change. An invited presentation to the New Zealand Emissions Trading Scheme Review Committee. Martin Manning, Andy Reisinger, David Wratt February 2009 About the Authors: Prof Manning was Director of the IPCC Working Group I Technical Support Unit based in Colorado from 2002 – 2007. He has also been an elected member of the IPCC Bureau, a Coordinating Lead Author, Contributing Author, and Review Editor for IPCC reports and a government delegate to IPCC Panel Sessions from 1992 to 2002. Dr Andy Reisinger was Head of the IPCC Synthesis Report Technical Support Unit based in the UK and India from 2006– 2008. He was the IPCC Focal Point and government delegate to IPCC Panel Sessions for New Zealand from 2001 - 2006. Dr David Wratt has been a member of the IPCC Working Group I Bureau for the fourth and fifth IPCC assessment rounds. He has also been a Coordinating Lead Author, and Review Editor for IPCC reports, and a New Zealand government delegate to IPCC Panel Sessions since 1995. Climate Change Research Institute 1. Overview This paper supports an invited presentation to the New Zealand Emissions Trading Scheme Review Committee on the IPCC process by the Climate Change Research Institute of Victoria University. -
Australia and New Zealand
12 Australia and New Zealand BARRIE PITTOCK (AUSTRALIA) AND DAVID WRATT (NEW ZEALAND) Lead Authors: R. Basher (New Zealand), B. Bates (Australia), M. Finlayson (Australia), H. Gitay (Australia), A. Woodward (New Zealand) Contributing Authors: A. Ar thington (Australia), P. Beets (New Zealand), B. Biggs (New Zealand), H. Clark (New Zealand), I. Cole (Australia), B. Collyer (Australia), S. Crimp (Australia), K. Day (Australia), J. Ford-Robertson (New Zealand), F. Ghassemi (Australia), J. Grieve (New Zealand), D. Griffin (Australia), A. Hall (New Zealand), W. Hall (Australia), G. Horgan (New Zealand), P.D. Jamieson (New Zealand), R. Jones (Australia), G. Kenny (New Zealand), S. Lake (Australia), R. Leigh (Australia), V. Lyne (Australia), M. McGlone (New Zealand), K. McInnes (Australia), G. McKeon (Australia), J. McKoy (New Zealand), B. Mullan (New Zealand), P. Newton (New Zealand), J. Renwick (New Zealand), D. Smith (Australia), B. Sutherst (Australia), K. Walsh (Australia), B. Watson (Australia), D. White (Australia), T. Yonow (Australia) Review Editor: M. Howden (Australia) CONTENTS Executive Summary 59 3 12 . 6 . Settlements and Industry 61 5 12 . 6 . 1 . Infrastructure 61 5 12 . 1 . The Australasian Region 59 5 12 . 6 . 2 . Investment and Insurance 61 7 12 . 1 . 1 . Ov e r v i e w 59 5 12 . 6 . 3 . En e r gy and Minerals 61 7 12 . 1 . 2 . Previous Wor k 59 5 12 . 6 . 4 . Coastal Development 12 . 1 . 3 . Socioeconomic Tre n d s 59 6 and Management, Tou r i s m 61 7 12 . 1 . 4 . Climate Tre n d s 59 6 12 . 6 . 5 . Risk Management 61 8 12 . -
Developing a Comprehensive Approach to Climate Change Policy in the United States: Integrating Levels of Government and Economic Sectors
PREPUBLICATION DRAFT To be published in the University of Virginia Environmental Law Review, not for publication elsewhere Developing a Comprehensive Approach to Climate Change Policy in the United States: Integrating Levels of Government and Economic Sectors Thomas D. Peterson,* Robert B. McKinstry, Jr.**, and John C. Dernbach*** Introduction In the past few years the issue of global warming has become a national political priority and will likely remain one of the United States’ and the world’s most pressing and unresolved policy issues for many years. Many factors underlie the call for action. These include: advancement of world science assessments; expansion of public awareness and news coverage; increased severe weather events; noticeable global warming trends; continued recalcitrance toward action by the federal government; international pressure related to treaty obligations; business successes in reducing emissions; businesses demands for a coherent long range national strategy; mounting national energy policy problems, a tidal wave of state and local leadership actions, and court actions. With the Supreme Court’s landmark decision in Massachusetts v. E.P.A.,1 the release of the Fourth Report of the Intergovernmental Panel on Climate Change,2 and several other notable actions in 2007 (including announcement of new state greenhouse gas (GHG) mitigation plans by key governors),3 many believe that a tipping point has been reached, where a mandatory and comprehensive federal response to climate change has become inevitable. The Supreme Court’s decision in Massachusetts v. E.P.A.4 also makes invevitable a national program to address climate change under the Clean Air Act (CAA).5 Reversing the Administration’s denial of a petition to regulate mobile source emissions under * Senior Research Associate at the Penn State Department of Geography and Adjunct Professor at the Dickinson-Penn State Law School. -
Download the Full 2011 Year in Review
Year in Review enhancing the benefits of New Zealand’s natural resources1 enhancing the benefits of New Zealand’s natural resources NIWA Year in Review Contents 2011 Chairman & Chief Executive’s Report 2 Financial summary 8 Organisational responsibility 10 Our science 12 Our people 42 Directory 52 From the top of the atmosphere to the bottom of the ocean, NIWA is focused on enhancing the benefits of New Zealand’s natural resources Front cover: NIWA’s iconic Undersea New Zealand graphically identifies New Zealand’s ocean estate, the fourth largest in the world. staff across 16 sites in revenue from research and current environmental This page: This NIWA chart reveals the shape of the seabed in Cook Strait, New Zealand and Australia applied science services science projects uncovering some of the secrets hidden beneath the ocean (see page 32). Contents 1 Chairman & Chief Executive’s Report Enhancing our nation’s economic “New Zealand’s economic and environmental prosperity and environmental prosperity depends on robust, long-term science and applied research.” John Morgan, Chief Executive Never before in New Zealand’s history has the case for robust, long-term natural resources and environmental research and applied science services been made clearer than in the last financial year. As a country, we continue to we will have to make as a country navigate our way through difficult around our natural resources and economic times, made worse by environment in the coming years. unprecedented national disasters, Despite the challenges of the past like the Christchurch earthquakes year, NIWA has continued to perform and the Pike River mining tragedy. -
Changes in Drought Risk with Climate Change
Changes in drought risk with climate change Brett Mullan Alan Porteous David Wratt Michele Hollis Prepared for Ministry for the Environment (NZ Climate Change Office) Ministry of Agriculture and Forestry NIWA Client Report: WLG2005-23 May 2005 NIWA Project: MFE05305 National Institute of Water & Atmospheric Research Ltd 301 Evans Bay Parade, Greta Point, Wellington Private Bag 14901, Kilbirnie, Wellington, New Zealand Phone +64-4-386 0300, Fax +64-4-386 0574 www.niwa.co.nz © All rights reserved. This publication may not be reproduced or copied in any form without the permission of the client. Such permission is to be given only in accordance with the terms of the client's contract with NIWA. This copyright extends to all forms of copying and any storage of material in any kind of information retrieval system. Executive Summary As human activity adds more greenhouse gas to the atmosphere, most climate change scenarios predict rising temperatures and decreased rainfall in the east of New Zealand. This means eastern parts of the country are expected to experience more droughts as the 21st century goes on. Our report seeks for the first time to define the possible range of changes in future drought risk. This report was commissioned because of the importance of drought for agriculture and water resources. The report aims to give central and local government and the agriculture sector an indication of how big future drought changes could be in the various regions. This information can be relevant in managing long-term water resources and land use, including planning for irrigation schemes. -
Meteorological Society of New Zealand (Inc.)
ISSN 0111-1736 Meteorological Society Of New Zealand (Inc.) NEWSLETTER 119 DECEMBER 2009 Meteorological Society of New Zealand Newsletter - December 2009 - Page 2 Meteorological Society Of New Zealand (Inc.) NEWSLETTER 119 DECEMBER 2009 PO Box 6523, Marion Square, Wellington 6141, New Zealand Please forward contributions to Bob McDavitt, [email protected] CONTENTS Page AGM and Financial Report 3-6 Regional Reports 7 2009 Met Soc conference 8-9 2010 NIWA UV Conference 10-12 Stink 12 Kids Weather on the Internet 13 Winter 2009 14-21 Winter – in the media 22-42 Your new Committee President Kim Dirks [email protected] Immediate Past President Mike Revell Auckland VP Jennifer Salmond [email protected] Wellington VP James Renwick [email protected] Christchurch VP Vacant Dunedin VP vacant Secretary Sam Dean [email protected] Treasurer Cliff Revell [email protected] Circulation Manager Sylvia Nichols [email protected] Journal Editor Brian Giles [email protected] Newsletter Editor Bob McDavitt [email protected] Wed Editor Peter Knudsen [email protected] Hydrological Society Liaison Charles Pearson [email protected] General Committee Jim Salinger [email protected] Stacey Dravitzki (to be confirmed) [email protected] Katrina Richards [email protected] Sally Garrett [email protected] Gareth Renowden [email protected] Simon Kjellberg Views and endorsements expressed in this newsletter are those of the contributors and advertisers, and not necessarily those of the Meteorological Society of New Zealand. The mention of specific companies or products does not imply that they are endorsed or recommended by the Society. -
Generating Multiyear Gridded Daily Rainfall Over New Zealand
SEPTEMBER 2005 T A I T A N D TURNER 1315 Generating Multiyear Gridded Daily Rainfall over New Zealand ANDREW TAIT AND RICHARD TURNER National Institute of Water and Atmospheric Research, Kilbirnie, Wellington, New Zealand (Manuscript received 14 June 2004, in final form 2 March 2005) ABSTRACT Daily rainfall totals are a key input for hydrological models that are designed to simulate water and pollutant flow through both soil and waterways. Within New Zealand there are large areas and many river catchments where no long-term rainfall observations exist. A method for estimating daily rainfall over the whole of New Zealand on a 5-km grid is described and tested over a period from January 1985 to April 2002. Improvement over a spatial interpolation method was gained by scaling high-elevation rainfall estimates using simulated mesoscale model rainfall surfaces that are generated for short periods in 1994 and 1996. This method is judged to produce reasonable and useful estimates of daily rainfall. 1. Introduction This study examines a method for estimating daily rainfall on a regular 5-km grid, covering all of New In recent years, hydrological models such as the Spa- Zealand for the period of 1985–2002, for the purpose of tially Referenced Regressions on Watershed Attributes input into and testing of hydrological models. The ap- (SPARROW; Brakebill and Preston 2003) have been proach combines daily rainfalls that are interpolated used to provide information about the effects of land- from climate station sites with short-period-simulated use change on water quality in rivers. In New Zealand, mesoscale model rainfall surfaces. -
Synthesis Report Synthesis Report
Climate Change 2007: Synthesis Report Synthesis Report An Assessment of the Intergovernmental Panel on Climate Change This underlying report, adopted section by section at IPCC Plenary XXVII (Valencia, Spain, 12-17 November 2007), represents the formally agreed statement of the IPCC concerning key findings and uncertainties contained in the Working Group contributions to the Fourth Assessment Report. Based on a draft prepared by: Core Writing Team Lenny Bernstein, Peter Bosch, Osvaldo Canziani, Zhenlin Chen, Renate Christ, Ogunlade Davidson, William Hare, Saleemul Huq, David Karoly, Vladimir Kattsov, Zbigniew Kundzewicz, Jian Liu, Ulrike Lohmann, Martin Manning, Taroh Matsuno, Bettina Menne, Bert Metz, Monirul Mirza, Neville Nicholls, Leonard Nurse, Rajendra Pachauri, Jean Palutikof, Martin Parry, Dahe Qin, Nijavalli Ravindranath, Andy Reisinger, Jiawen Ren, Keywan Riahi, Cynthia Rosenzweig, Matilde Rusticucci, Stephen Schneider, Youba Sokona, Susan Solomon, Peter Stott, Ronald Stouffer, Taishi Sugiyama, Rob Swart, Dennis Tirpak, Coleen Vogel, Gary Yohe Extended Writing Team Terry Barker Review Editors Abdelkader Allali, Roxana Bojariu, Sandra Diaz, Ismail Elgizouli, Dave Griggs, David Hawkins, Olav Hohmeyer, Bubu Pateh Jallow, Luc 4ka Kajfez4-Bogataj, Neil Leary, Hoesung Lee, David Wratt Introduction Introduction ment. Topic 5 assesses the relationship between adaptation and Introduction mitigation on a more conceptual basis and takes a longer-term per- spective. Topic 6 summarises the major robust findings and remain- This Synthesis Report is based on the assessment carried out ing key uncertainties in this assessment. by the three Working Groups (WGs) of the Intergovernmental Panel A schematic framework representing anthropogenic drivers, on Climate Change (IPCC). It provides an integrated view of cli- impacts of and responses to climate change, and their linkages, is mate change as the final part of the IPCC’s Fourth Assessment Re- shown in Figure I.1. -
Strengthening Climate Services in Samoa FINAL
Integrating Climate Change Risk in the Agriculture and Health Sectors in Samoa (ICCRAHS) STRENGTHENING CLIMATE SERVICES IN SAMOA Recommendations for the next development phase of integrating climate change mitigation and adaptation services into the agriculture and health sectors in Samoa [2013─2018] Compiled for the Ministry of Natural Resources and Environment Government of Samoa & United Nations Development Programme February 2013 Contributors: Alan Porteous, Andrew Tait, Austealia Titimaea, Sunny Seuseu, Doug Ramsay, Penehuro Lefale, David Wratt, Teddy Allen, Marta Moneo. Corresponding editor: Alan Porteous NIWA (National Institute of Water and Atmospheric Research) PO Box 14901 Wellington New Zealand. [email protected] Page 2 Version 4, February 2013 EXECUTIVE SUMMARY The current phase of the implementation of the GEF- funded project, ‘Integrating climate change risk in the Agriculture and Health Sectors in Samoa’ (ICCRAHS), managed by the Samoan Ministry of Natural Resources and Environment (MNRE), ends in 2013. Planning is under way to build on the outcomes from this project to further develop climate services in Samoa. This document, requested by MNRE and UNDP, draws on two sources of guidance to evaluate the progress of climate services so far in Samoa, and to point to planning and implementation priorities: • needs and gaps in current climate services expressed by state sector workers and other stakeholders at a Sector Engagement Workshop in Apia in October 2012; • the Global Framework for Climate Services (GFCS) Implementation Plan endorsed at the Extraordinary Session of the WMO Congress (Cg-Ext (2012)) in November 2012, Significant progress has been achieved during the implementation of ICCRAHS to enhance the capacity of the Samoa Meteorological Division (SMD) to observe, capture and manage weather and climate data. -
Curriculum Vitae Dr. Howard J. Diamond NOAA/National Climatic
Curriculum Vitae Dr. Howard J. Diamond NOAA/National Climatic Data Center 1100 Wayne Avenue, Suite 1202 Silver Spring, Maryland United States of America Mailing Code: 20910 Tel: +1-301-427-2475 (Work) Fax: +1-301-427-0033 Email: [email protected] or [email protected] or [email protected] Personal Statement: Howard Diamond has worked in various positions within the National Oceanic and Atmospheric Administration (NOAA) since 1981 and currently works for NOAA’s National Climatic Data Center (NCDC) in Silver Spring, Maryland. In 1999, Dr. Diamond began serving as the U.S. Global Climate Observing System Program Manager. Since then he assumed the roles as (a) editor of the Tropics Chapter for the annual State of the Climate reports coordinated and published by NCDC (since 2005); (b) Director of the World Data Center for Meteorology (since 2006); (c) program manager for the U.S. Climate Reference Network program (since 2007); (d) atmospheric climate observing liaison to the NOAA Climate Program Office’s Climate Observations Division (since 2007); and (e) member of the International Best Tracks Archive for Climate Stewardship (IBTrACS) science and product team (since 2009). In these various positions he has concentrated his efforts in implementing sustained climate observing programs (and related data management efforts) both in the U.S., as well as in developing nations with a particular emphasis in the Pacific Islands region. In this regard he has worked closely with many individuals from several organizations from many nations, and particularly from New Zealand and Australia. His specific research areas of interest involve southern hemispheric climate research involving tropical cyclones (including assisting in the production of the annual TC outlook for the southwest Pacific), Antarctic sea ice, and general trends in climate that involve relationships to various global and regional teleconnections such as the El Niño Southern Oscillation, Southern Annular Mode, Pacific Decadal Oscillation, and Madden-Julian Oscillation. -
Global Warming: Forecasts by Scientists Versus Scientific Forecasts
University of Pennsylvania ScholarlyCommons Marketing Papers Wharton Faculty Research 2007 Global Warming: Forecasts by Scientists versus Scientific Forecasts Kesten C. Green Monash University, [email protected] J. Scott Armstrong University of Pennsylvania, [email protected] Follow this and additional works at: https://repository.upenn.edu/marketing_papers Recommended Citation Green, K. C., & Armstrong, J. S. (2007). Global Warming: Forecasts by Scientists versus Scientific Forecasts. Retrieved from https://repository.upenn.edu/marketing_papers/168 Suggested Citation: Green, K.C. and Armstrong, J.S. (2007). Global Warming: Forecasts by Scienctists Versus Scientific Forecasts. Energy & Environment. Vol. 19(7-8). p. 997-1021. Publisher URL: http://multi-science.metapress.com/content/121493/ This paper is posted at ScholarlyCommons. https://repository.upenn.edu/marketing_papers/168 For more information, please contact [email protected]. Global Warming: Forecasts by Scientists versus Scientific orF ecasts Abstract In 2007, the Intergovernmental Panel on Climate Change’s Working Group One, a panel of experts established by the World Meteorological Organization and the United Nations Environment Programme, issued its Fourth Assessment Report. The Report included predictions of dramatic increases in average world temperatures over the next 92 years and serious harm resulting from the predicted temperature increases. Using forecasting principles as our guide we asked: Are these forecasts a good basis for developing public policy? Our answer is “no”. To provide forecasts of climate change that are useful for policy-making, one would need to forecast (1) global temperature, (2) the effects of any temperature changes, and (3) the effects of feasible alternative policies. Proper forecasts of all three are necessary for rational policy making.