Mechanisms of Nmda Receptor Inhibition by Memantine and Ketamine
Total Page:16
File Type:pdf, Size:1020Kb
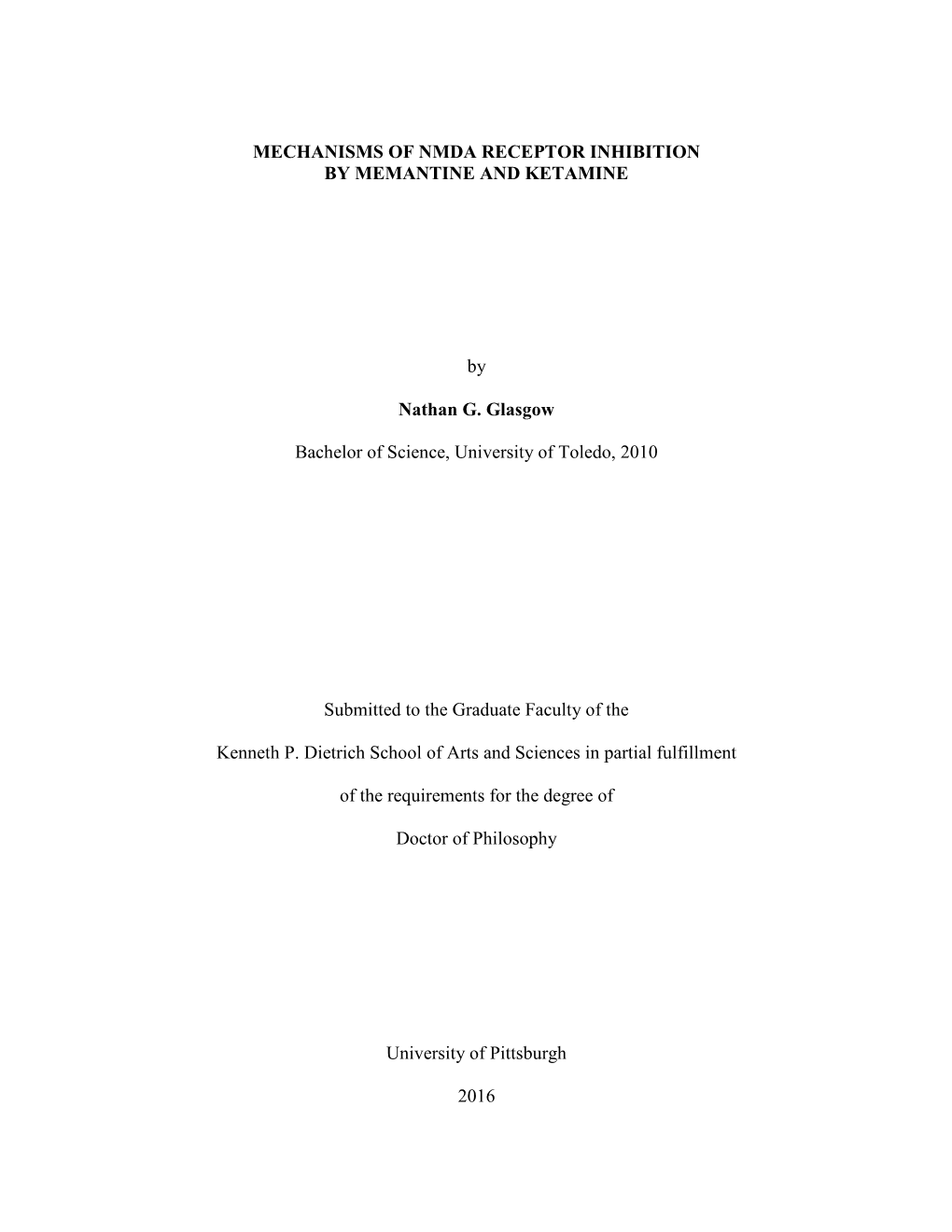
Load more
Recommended publications
-
Opioid-Induced Hyperalgesia in Humans Molecular Mechanisms and Clinical Considerations
SPECIAL TOPIC SERIES Opioid-induced Hyperalgesia in Humans Molecular Mechanisms and Clinical Considerations Larry F. Chu, MD, MS (BCHM), MS (Epidemiology),* Martin S. Angst, MD,* and David Clark, MD, PhD*w treatment of acute and cancer-related pain. However, Abstract: Opioid-induced hyperalgesia (OIH) is most broadly recent evidence suggests that opioid medications may also defined as a state of nociceptive sensitization caused by exposure be useful for the treatment of chronic noncancer pain, at to opioids. The state is characterized by a paradoxical response least in the short term.3–14 whereby a patient receiving opioids for the treatment of pain Perhaps because of this new evidence, opioid may actually become more sensitive to certain painful stimuli. medications have been increasingly prescribed by primary The type of pain experienced may or may not be different from care physicians and other patient care providers for the original underlying painful condition. Although the precise chronic painful conditions.15,16 Indeed, opioids are molecular mechanism is not yet understood, it is generally among the most common medications prescribed by thought to result from neuroplastic changes in the peripheral physicians in the United States17 and accounted for 235 and central nervous systems that lead to sensitization of million prescriptions in the year 2004.18 pronociceptive pathways. OIH seems to be a distinct, definable, One of the principal factors that differentiate the use and characteristic phenomenon that may explain loss of opioid of opioids for the treatment of pain concerns the duration efficacy in some cases. Clinicians should suspect expression of of intended use. -
Citizen Cyborg.” Citizen a Groundbreaking Work of Social Commentary, Citizen Cyborg Artificial Intelligence, Nanotechnology, and Genetic Engineering —DR
hughes (continued from front flap) $26.95 US ADVANCE PRAISE FOR ARTIFICIAL INTELLIGENCE NANOTECHNOLOGY GENETIC ENGINEERING MEDICAL ETHICS INVITRO FERTILIZATION STEM-CELL RESEARCH $37.95 CAN citizen LIFE EXTENSION GENETIC PATENTS HUMAN GENETIC ENGINEERING CLONING SEX SELECTION ASSISTED SUICIDE UNIVERSAL HEALTHCARE human genetic engineering, sex selection, drugs, and assisted In the next fifty years, life spans will extend well beyond a century. suicide—and concludes with a concrete political agenda for pro- cyborg Our senses and cognition will be enhanced. We will have greater technology progressives, including expanding and deepening control over our emotions and memory. Our bodies and brains “A challenging and provocative look at the intersection of human self-modification and human rights, reforming genetic patent laws, and providing SOCIETIES MUST RESPOND TO THE REDESIGNED HUMAN OF FUTURE WHY DEMOCRATIC will be surrounded by and merged with computer power. The limits political governance. Everyone wondering how society will be able to handle the coming citizen everyone with healthcare and a basic guaranteed income. of the human body will be transcended, as technologies such as possibilities of A.I. and genomics should read Citizen Cyborg.” citizen A groundbreaking work of social commentary, Citizen Cyborg artificial intelligence, nanotechnology, and genetic engineering —DR. GREGORY STOCK, author of Redesigning Humans illuminates the technologies that are pushing the boundaries of converge and accelerate. With them, we will redesign ourselves and humanness—and the debate that may determine the future of the our children into varieties of posthumanity. “A powerful indictment of the anti-rationalist attitudes that are dominating our national human race itself. -
Addictions and the Brain
9/18/2012 Addictions and the Brain TAAP Conference September 14, 2012 Acknowledgements • La Hacienda Treatment Center • American Society of Addiction Medicine • National Institute of Drug Abuse © 2012 La Hacienda Treatment Center. All rights reserved. 1 9/18/2012 Definition • A primary, progressive biochemical, psychosocial, genetically transmitted chronic disease of relapse who’s hallmarks are denial, loss of control and unmanageability. DSM IV Criteria for dependency: At least 3 of the 7 below 1. Withdrawal 2. Tolerance 3. The substance is taken in larger amounts or over a longer period than was intended. 4. There is a persistent desire or unsuccessful efforts to cut down or control substance use. 5. A great deal of time is spent in activities necessary to obtain the substance, use the substance, or recover from its effects. 6. Important social, occupational, or recreational activities are given up or reduced because of the substance use. 7. The substance use is continued despite knowledge of having a persistent or recurrent physical or psychological problem that is likely to have been caused or exacerbated by the substance. © 2012 La Hacienda Treatment Center. All rights reserved. 2 9/18/2012 Dispute between behavior and disease Present understanding of the Hypothalamus location of the disease hypothesis. © 2012 La Hacienda Treatment Center. All rights reserved. 3 9/18/2012 © 2012 La Hacienda Treatment Center. All rights reserved. 4 9/18/2012 © 2012 La Hacienda Treatment Center. All rights reserved. 5 9/18/2012 Dispute regarding behavior versus disease © 2012 La Hacienda Treatment Center. All rights reserved. 6 9/18/2012 © 2012 La Hacienda Treatment Center. -
Role of a Hippocampal Src-Family Kinase-Mediated Glutamatergic Mechanism in Drug Context-Induced Cocaine Seeking
Neuropsychopharmacology (2013) 38, 2657–2665 & 2013 American College of Neuropsychopharmacology. All rights reserved 0893-133X/13 www.neuropsychopharmacology.org Role of a Hippocampal Src-Family Kinase-Mediated Glutamatergic Mechanism in Drug Context-Induced Cocaine Seeking 1 1 1 1 ,1 Xiaohu Xie , Amy A Arguello , Audrey M Wells , Andrew M Reittinger and Rita A Fuchs* 1 Department of Psychology, University of North Carolina, Chapel Hill, NC, USA Glutamatergic neurotransmission in the dorsal hippocampus (DH) is necessary for drug context-induced reinstatement of cocaine- seeking behavior in an animal model of drug relapse. Furthermore, in vitro studies suggest that the Src family of tyrosine kinases critically regulates glutamatergic cellular functions within the DH. Thus, Src-family kinases in the DH may similarly control contextual cocaine- seeking behavior. To test this hypothesis, rats were trained to lever press for un-signaled cocaine infusions in a distinct context followed by extinction training in a different context. Cocaine-seeking behavior (non-reinforced active lever pressing) was then assessed in the previously cocaine-paired and extinction contexts after AP5 (N-methyl-D-aspartate glutamate (NMDA) receptor (NMDAR) antagonist; 0.25 or 2.5 mg/0.5 ml/hemisphere), PP2 (Src-family kinase inhibitor; 6.25 or 62.5 ng/0.5 ml/hemisphere), Ro25-6981 (NR2B subunit- containing NMDAR antagonist; 0.2 or 2 mg/0.5 ml/hemisphere), or vehicle administration into the DH. Administration of AP5, PP2, or Ro25-6981 into the DH dose-dependently impaired drug context-induced reinstatement of cocaine-seeking behavior relative to vehicle, without altering instrumental behavior in the extinction context or food-reinforced instrumental responding and general motor activity in control experiments. -
“STOP” and “GO” Pathways for the Treatment of Alcohol Use Disorders
UCSF UC San Francisco Previously Published Works Title Targeting the intracellular signaling "STOP" and "GO" pathways for the treatment of alcohol use disorders. Permalink https://escholarship.org/uc/item/6hd3x2cv Journal Psychopharmacology, 235(6) ISSN 0033-3158 Authors Ron, Dorit Berger, Anthony Publication Date 2018-06-01 DOI 10.1007/s00213-018-4882-z Peer reviewed eScholarship.org Powered by the California Digital Library University of California Psychopharmacology (2018) 235:1727–1743 https://doi.org/10.1007/s00213-018-4882-z REVIEW Targeting the intracellular signaling “STOP” and “GO” pathways for the treatment of alcohol use disorders Dorit Ron1 & Anthony Berger1 Received: 18 January 2018 /Accepted: 12 March 2018 /Published online: 14 April 2018 # The Author(s) 2018 Abstract In recent years, research has identified the molecular and neural substrates underlying the transition of moderate “social” con- sumption of alcohol to the characteristic alcohol use disorder (AUD) phenotypes including excessive and compulsive alcohol use which we define in the review as the GO signaling pathways. In addition, growing evidence points to the existence of molecular mechanisms that keep alcohol consumption in check and that confer resilience for the development of AUD which we define herein as the STOP signaling pathways. In this review, we focus on examples of the GO and the STOP intracellular signaling pathways and discuss our current knowledge of how manipulations of these pathways may be used for the treatment of AUD. Keywords Alcohol . Addiction . Signaling . Translation . Medication Development . Fyn . mTOR . BDNF . GDNF Introduction medications such as naltrexone, acamprosate, and disulfiram not only are beneficial but also suffer from efficacy and com- Alcohol use disorder (AUD) is a serious worldwide health prob- pliance issues (Wackernah et al. -
Tinnitus Hopes Put to Sleep by Latest Auris Failure
March 14, 2018 Tinnitus hopes put to sleep by latest Auris failure Madeleine Armstrong Ketamine is best known as a horse tranquiliser or a recreational drug, but it has also been proposed as a treatment for various disorders from depression to Alzheimer’s. Hopes of developing the drug in tinnitus have been dashed by the failure of Auris’s Keyzilen in a second phase III trial. As well as leaving Auris’s future looking bleak – Keyzilen is the second of its phase III candidates to flunk in four months – the setback could also be bad news for the sparse tinnitus pipeline. According to EvaluatePharma there is only one other candidate in active clinical development, Otonomy’s OTO-313, and this uses the same mechanism of action as Keyzilen (see table below). Tinnitus pipeline Generic Project Company Pharma class Trial(s) Notes name Phase III Auris Esketamine TACTT2 Keyzilen NMDA antagonist Failed Aug 2016 Medical hydrochloride (NCT01803646) TACTT3 Failed Mar 2018 (NCT02040194) Phase I OTO- Phase I/II trial to OTO-311 abandoned in Otonomy NMDA antagonist Gacyclidine 313 start H1 2019 favour of this formulation Preclinical Auris AM-102 Undisclosed - - Medical KCNQ2 Knopp Kv7 potassium - - Program Biosciences channel modulator Source: EvaluatePharma. Both projects are psychoactive drugs targeting the NMDA receptor. Tinnitus is commonly caused by loud noise and resulting damage to the sensory hair cells in the cochlea. Initial trauma to the inner ear has been shown to trigger excess production of glutamate, which leads to the hyperactivation of NMDA receptors and, in turn, cell death. Blocking the NMDA receptor could therefore have a protective effect – but it is unclear how this mechanism would work once the damage to hair cells had been done. -
Interplay Between Gating and Block of Ligand-Gated Ion Channels
brain sciences Review Interplay between Gating and Block of Ligand-Gated Ion Channels Matthew B. Phillips 1,2, Aparna Nigam 1 and Jon W. Johnson 1,2,* 1 Department of Neuroscience, University of Pittsburgh, Pittsburgh, PA 15260, USA; [email protected] (M.B.P.); [email protected] (A.N.) 2 Center for Neuroscience, University of Pittsburgh, Pittsburgh, PA 15260, USA * Correspondence: [email protected]; Tel.: +1-(412)-624-4295 Received: 27 October 2020; Accepted: 26 November 2020; Published: 1 December 2020 Abstract: Drugs that inhibit ion channel function by binding in the channel and preventing current flow, known as channel blockers, can be used as powerful tools for analysis of channel properties. Channel blockers are used to probe both the sophisticated structure and basic biophysical properties of ion channels. Gating, the mechanism that controls the opening and closing of ion channels, can be profoundly influenced by channel blocking drugs. Channel block and gating are reciprocally connected; gating controls access of channel blockers to their binding sites, and channel-blocking drugs can have profound and diverse effects on the rates of gating transitions and on the stability of channel open and closed states. This review synthesizes knowledge of the inherent intertwining of block and gating of excitatory ligand-gated ion channels, with a focus on the utility of channel blockers as analytic probes of ionotropic glutamate receptor channel function. Keywords: ligand-gated ion channel; channel block; channel gating; nicotinic acetylcholine receptor; ionotropic glutamate receptor; AMPA receptor; kainate receptor; NMDA receptor 1. Introduction Neuronal information processing depends on the distribution and properties of the ion channels found in neuronal membranes. -
From NMDA Receptor Hypofunction to the Dopamine Hypothesis of Schizophrenia J
REVIEW The Neuropsychopharmacology of Phencyclidine: From NMDA Receptor Hypofunction to the Dopamine Hypothesis of Schizophrenia J. David Jentsch, Ph.D., and Robert H. Roth, Ph.D. Administration of noncompetitive NMDA/glutamate effects of these drugs are discussed, especially with regard to receptor antagonists, such as phencyclidine (PCP) and differing profiles following single-dose and long-term ketamine, to humans induces a broad range of exposure. The neurochemical effects of NMDA receptor schizophrenic-like symptomatology, findings that have antagonist administration are argued to support a contributed to a hypoglutamatergic hypothesis of neurobiological hypothesis of schizophrenia, which includes schizophrenia. Moreover, a history of experimental pathophysiology within several neurotransmitter systems, investigations of the effects of these drugs in animals manifested in behavioral pathology. Future directions for suggests that NMDA receptor antagonists may model some the application of NMDA receptor antagonist models of behavioral symptoms of schizophrenia in nonhuman schizophrenia to preclinical and pathophysiological research subjects. In this review, the usefulness of PCP are offered. [Neuropsychopharmacology 20:201–225, administration as a potential animal model of schizophrenia 1999] © 1999 American College of is considered. To support the contention that NMDA Neuropsychopharmacology. Published by Elsevier receptor antagonist administration represents a viable Science Inc. model of schizophrenia, the behavioral and neurobiological KEY WORDS: Ketamine; Phencyclidine; Psychotomimetic; widely from the administration of purportedly psychot- Memory; Catecholamine; Schizophrenia; Prefrontal cortex; omimetic drugs (Snyder 1988; Javitt and Zukin 1991; Cognition; Dopamine; Glutamate Jentsch et al. 1998a), to perinatal insults (Lipska et al. Biological psychiatric research has seen the develop- 1993; El-Khodor and Boksa 1997; Moore and Grace ment of many putative animal models of schizophrenia. -
NINDS Custom Collection II
ACACETIN ACEBUTOLOL HYDROCHLORIDE ACECLIDINE HYDROCHLORIDE ACEMETACIN ACETAMINOPHEN ACETAMINOSALOL ACETANILIDE ACETARSOL ACETAZOLAMIDE ACETOHYDROXAMIC ACID ACETRIAZOIC ACID ACETYL TYROSINE ETHYL ESTER ACETYLCARNITINE ACETYLCHOLINE ACETYLCYSTEINE ACETYLGLUCOSAMINE ACETYLGLUTAMIC ACID ACETYL-L-LEUCINE ACETYLPHENYLALANINE ACETYLSEROTONIN ACETYLTRYPTOPHAN ACEXAMIC ACID ACIVICIN ACLACINOMYCIN A1 ACONITINE ACRIFLAVINIUM HYDROCHLORIDE ACRISORCIN ACTINONIN ACYCLOVIR ADENOSINE PHOSPHATE ADENOSINE ADRENALINE BITARTRATE AESCULIN AJMALINE AKLAVINE HYDROCHLORIDE ALANYL-dl-LEUCINE ALANYL-dl-PHENYLALANINE ALAPROCLATE ALBENDAZOLE ALBUTEROL ALEXIDINE HYDROCHLORIDE ALLANTOIN ALLOPURINOL ALMOTRIPTAN ALOIN ALPRENOLOL ALTRETAMINE ALVERINE CITRATE AMANTADINE HYDROCHLORIDE AMBROXOL HYDROCHLORIDE AMCINONIDE AMIKACIN SULFATE AMILORIDE HYDROCHLORIDE 3-AMINOBENZAMIDE gamma-AMINOBUTYRIC ACID AMINOCAPROIC ACID N- (2-AMINOETHYL)-4-CHLOROBENZAMIDE (RO-16-6491) AMINOGLUTETHIMIDE AMINOHIPPURIC ACID AMINOHYDROXYBUTYRIC ACID AMINOLEVULINIC ACID HYDROCHLORIDE AMINOPHENAZONE 3-AMINOPROPANESULPHONIC ACID AMINOPYRIDINE 9-AMINO-1,2,3,4-TETRAHYDROACRIDINE HYDROCHLORIDE AMINOTHIAZOLE AMIODARONE HYDROCHLORIDE AMIPRILOSE AMITRIPTYLINE HYDROCHLORIDE AMLODIPINE BESYLATE AMODIAQUINE DIHYDROCHLORIDE AMOXEPINE AMOXICILLIN AMPICILLIN SODIUM AMPROLIUM AMRINONE AMYGDALIN ANABASAMINE HYDROCHLORIDE ANABASINE HYDROCHLORIDE ANCITABINE HYDROCHLORIDE ANDROSTERONE SODIUM SULFATE ANIRACETAM ANISINDIONE ANISODAMINE ANISOMYCIN ANTAZOLINE PHOSPHATE ANTHRALIN ANTIMYCIN A (A1 shown) ANTIPYRINE APHYLLIC -
Aniracetam Reduces Glutamate Receptor Desensitization and Slows
Proc. Natl. Acad. Sci. USA Vol. 88, pp. 10936-10940, December 1991 Neurobiology Aniracetam reduces glutamate receptor desensitization and slows the decay of fast excitatory synaptic currents in the hippocampus (non-N-methyl-D-aspartate receptor/synapse) JEFFRY S. ISAACSON*t AND ROGER A. NICOLLtt *Physiology Graduate Program and the Departments of SPharmacology and tPhysiology, University of California, San Francisco, CA 94143-0450 Communicated by Floyd E. Bloom, September 16, 1991 ABSTRACT Aniracetam is a nootropic drug that has been and DL-2-amino-5-phosphonovaleric acid (50 ,uM) were shown to selectively enhance quisqualate receptor-mediated added to the medium to block y-aminobutyric acid type A responses inXenopus oocytes injected with brain mRNA and in (GABAA) receptors and NMDA receptors, respectively. In hippocampal pyramidal cells [Ito, I., Tanabe, S., Kohda, A. & the majority of experiments examining iontophoretic re- Sugiyama, H. (1990) J. Physiol. (London) 424, 533-544]. We sponses, tetrodotoxin (0.5-1 1uM) was included to block have used patch clamp recording techniques in hippocampal sodium-dependent action potentials. Currents were recorded slices to elucidate the mechanism for this selective action. We with an Axopatch 1B amplifier from neurons in the CA1 and find that aniracetam enhances glutamate-evoked currents in CA3 pyramidal cell layers and granule cell layer of the whole-cell recordings and, in outside-out patches, strongly dentate gyrus using the "blind" whole-cell recording tech- reduces glutamate receptor desensitization. In addition, nique (15, 16). Patch electrodes (tip diameter = 2 Ium) aniracetam selectively prolongs the time course and increases contained (in mM) either a CsF (110 CsF, 10 CsCl, 10 Hepes, the peak amplitude of fast synaptic currents. -
Memantine Increases NMDA Receptor Level in the Prefrontal Cortex
View metadata, citation and similar papers at core.ac.uk brought to you by CORE provided by University of Dundee Online Publications University of Dundee Memantine increases NMDA receptor level in the prefrontal cortex but fails to reverse apomorphine-induced conditioned place preference in rats Ndlazi, Ziphozethu; Abboussi, Oualid; Mabandla, Musa; Daniels, Willie Published in: AIMS Neuroscience DOI: 10.3934/NEUROSCIENCE.2018.4.211 Publication date: 2018 Document Version Publisher's PDF, also known as Version of record Link to publication in Discovery Research Portal Citation for published version (APA): Ndlazi, Z., Abboussi, O., Mabandla, M., & Daniels, W. (2018). Memantine increases NMDA receptor level in the prefrontal cortex but fails to reverse apomorphine-induced conditioned place preference in rats. AIMS Neuroscience, 5(4), 211-220. https://doi.org/10.3934/NEUROSCIENCE.2018.4.211 General rights Copyright and moral rights for the publications made accessible in Discovery Research Portal are retained by the authors and/or other copyright owners and it is a condition of accessing publications that users recognise and abide by the legal requirements associated with these rights. • Users may download and print one copy of any publication from Discovery Research Portal for the purpose of private study or research. • You may not further distribute the material or use it for any profit-making activity or commercial gain. • You may freely distribute the URL identifying the publication in the public portal. Take down policy If you believe that this document breaches copyright please contact us providing details, and we will remove access to the work immediately and investigate your claim. -
Differential Effects of Extended Exercise and Memantine Treatment on Adult Neurogenesis in Male and Female Rats
bioRxiv preprint doi: https://doi.org/10.1101/332890; this version posted May 29, 2018. The copyright holder for this preprint (which was not certified by peer review) is the author/funder, who has granted bioRxiv a license to display the preprint in perpetuity. It is made available under aCC-BY-NC 4.0 International license. DIFFERENTIAL EFFECTS OF EXTENDED EXERCISE AND MEMANTINE TREATMENT ON ADULT NEUROGENESIS IN MALE AND FEMALE RATS Shaina P Cahill, John Darby Cole, Ru Qi Yu, Jack Clemans-Gibbon, Jason S Snyder* Department of Psychology Djavad Mowafaghian Centre for Brain Health University of British Columbia Vancouver, BC, Canada *Corresponding author email: [email protected] bioRxiv preprint doi: https://doi.org/10.1101/332890; this version posted May 29, 2018. The copyright holder for this preprint (which was not certified by peer review) is the author/funder, who has granted bioRxiv a license to display the preprint in perpetuity. It is made available under aCC-BY-NC 4.0 International license. ABSTRACT The creation of new neurons in adulthood has potential for treating a number of disorders that are characterized by neurodegeneration or impaired plasticity. Animal models of reduced neurogenesis, and studies of the volume and structural integrity of the hippocampus in humans, suggest a possible therapeutic role for adult neurogenesis in age-related cognitive decline, depression, and schizophrenia. Research over the past 20 years has identified a number of approaches for enhancing adult neurogenesis, such as exercise, NMDA receptor antagonists, antidepressant drugs and environmental enrichment. However, despite the chronic nature of many disorders that impact the human hippocampus, most animal studies have only examined the efficacy of neurogenic treatments over relatively short timescales (~1 month or less).