The Informational Substrate of Chemical Evolution: Implications for Abiogenesis
Total Page:16
File Type:pdf, Size:1020Kb
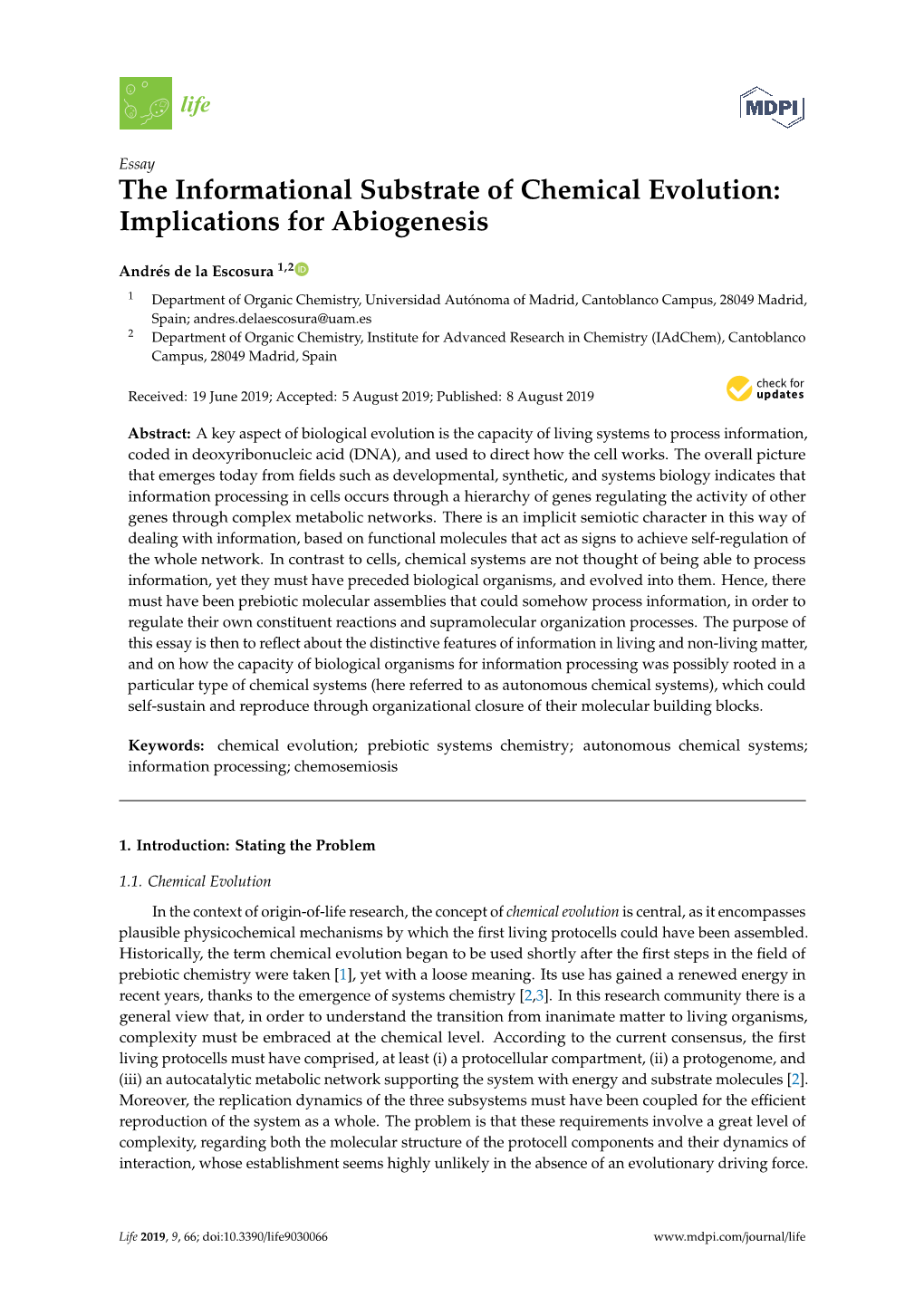
Load more
Recommended publications
-
Glossary - Cellbiology
1 Glossary - Cellbiology Blotting: (Blot Analysis) Widely used biochemical technique for detecting the presence of specific macromolecules (proteins, mRNAs, or DNA sequences) in a mixture. A sample first is separated on an agarose or polyacrylamide gel usually under denaturing conditions; the separated components are transferred (blotting) to a nitrocellulose sheet, which is exposed to a radiolabeled molecule that specifically binds to the macromolecule of interest, and then subjected to autoradiography. Northern B.: mRNAs are detected with a complementary DNA; Southern B.: DNA restriction fragments are detected with complementary nucleotide sequences; Western B.: Proteins are detected by specific antibodies. Cell: The fundamental unit of living organisms. Cells are bounded by a lipid-containing plasma membrane, containing the central nucleus, and the cytoplasm. Cells are generally capable of independent reproduction. More complex cells like Eukaryotes have various compartments (organelles) where special tasks essential for the survival of the cell take place. Cytoplasm: Viscous contents of a cell that are contained within the plasma membrane but, in eukaryotic cells, outside the nucleus. The part of the cytoplasm not contained in any organelle is called the Cytosol. Cytoskeleton: (Gk. ) Three dimensional network of fibrous elements, allowing precisely regulated movements of cell parts, transport organelles, and help to maintain a cell’s shape. • Actin filament: (Microfilaments) Ubiquitous eukaryotic cytoskeletal proteins (one end is attached to the cell-cortex) of two “twisted“ actin monomers; are important in the structural support and movement of cells. Each actin filament (F-actin) consists of two strands of globular subunits (G-Actin) wrapped around each other to form a polarized unit (high ionic cytoplasm lead to the formation of AF, whereas low ion-concentration disassembles AF). -
Chemistry Grade Level 10 Units 1-15
COPPELL ISD SUBJECT YEAR AT A GLANCE GRADE HEMISTRY UNITS C LEVEL 1-15 10 Program Transfer Goals ● Ask questions, recognize and define problems, and propose solutions. ● Safely and ethically collect, analyze, and evaluate appropriate data. ● Utilize, create, and analyze models to understand the world. ● Make valid claims and informed decisions based on scientific evidence. ● Effectively communicate scientific reasoning to a target audience. PACING 1st 9 Weeks 2nd 9 Weeks 3rd 9 Weeks 4th 9 Weeks Unit 1 Unit 2 Unit 3 Unit 4 Unit 5 Unit 6 Unit Unit Unit Unit Unit Unit Unit Unit Unit 7 8 9 10 11 12 13 14 15 1.5 wks 2 wks 1.5 wks 2 wks 3 wks 5.5 wks 1.5 2 2.5 2 wks 2 2 2 wks 1.5 1.5 wks wks wks wks wks wks wks Assurances for a Guaranteed and Viable Curriculum Adherence to this scope and sequence affords every member of the learning community clarity on the knowledge and skills on which each learner should demonstrate proficiency. In order to deliver a guaranteed and viable curriculum, our team commits to and ensures the following understandings: Shared Accountability: Responding -
Prebiological Evolution and the Metabolic Origins of Life
Prebiological Evolution and the Andrew J. Pratt* Metabolic Origins of Life University of Canterbury Keywords Abiogenesis, origin of life, metabolism, hydrothermal, iron Abstract The chemoton model of cells posits three subsystems: metabolism, compartmentalization, and information. A specific model for the prebiological evolution of a reproducing system with rudimentary versions of these three interdependent subsystems is presented. This is based on the initial emergence and reproduction of autocatalytic networks in hydrothermal microcompartments containing iron sulfide. The driving force for life was catalysis of the dissipation of the intrinsic redox gradient of the planet. The codependence of life on iron and phosphate provides chemical constraints on the ordering of prebiological evolution. The initial protometabolism was based on positive feedback loops associated with in situ carbon fixation in which the initial protometabolites modified the catalytic capacity and mobility of metal-based catalysts, especially iron-sulfur centers. A number of selection mechanisms, including catalytic efficiency and specificity, hydrolytic stability, and selective solubilization, are proposed as key determinants for autocatalytic reproduction exploited in protometabolic evolution. This evolutionary process led from autocatalytic networks within preexisting compartments to discrete, reproducing, mobile vesicular protocells with the capacity to use soluble sugar phosphates and hence the opportunity to develop nucleic acids. Fidelity of information transfer in the reproduction of these increasingly complex autocatalytic networks is a key selection pressure in prebiological evolution that eventually leads to the selection of nucleic acids as a digital information subsystem and hence the emergence of fully functional chemotons capable of Darwinian evolution. 1 Introduction: Chemoton Subsystems and Evolutionary Pathways Living cells are autocatalytic entities that harness redox energy via the selective catalysis of biochemical transformations. -
The Practice of Chemistry Education (Paper)
CHEMISTRY EDUCATION: THE PRACTICE OF CHEMISTRY EDUCATION RESEARCH AND PRACTICE (PAPER) 2004, Vol. 5, No. 1, pp. 69-87 Concept teaching and learning/ History and philosophy of science (HPS) Juan QUÍLEZ IES José Ballester, Departamento de Física y Química, Valencia (Spain) A HISTORICAL APPROACH TO THE DEVELOPMENT OF CHEMICAL EQUILIBRIUM THROUGH THE EVOLUTION OF THE AFFINITY CONCEPT: SOME EDUCATIONAL SUGGESTIONS Received 20 September 2003; revised 11 February 2004; in final form/accepted 20 February 2004 ABSTRACT: Three basic ideas should be considered when teaching and learning chemical equilibrium: incomplete reaction, reversibility and dynamics. In this study, we concentrate on how these three ideas have eventually defined the chemical equilibrium concept. To this end, we analyse the contexts of scientific inquiry that have allowed the growth of chemical equilibrium from the first ideas of chemical affinity. At the beginning of the 18th century, chemists began the construction of different affinity tables, based on the concept of elective affinities. Berthollet reworked this idea, considering that the amount of the substances involved in a reaction was a key factor accounting for the chemical forces. Guldberg and Waage attempted to measure those forces, formulating the first affinity mathematical equations. Finally, the first ideas providing a molecular interpretation of the macroscopic properties of equilibrium reactions were presented. The historical approach of the first key ideas may serve as a basis for an appropriate sequencing of -
Practical Resources for Enhancing the Reproducibility of Mechanistic Modeling in Systems Biology
Practical Resources for Enhancing the Reproducibility of Mechanistic Modeling in Systems Biology Michael L. Blinova,g, John H. Gennarib,g, Jonathan R. Karrc,d,g, Ion I. Morarua,g, David P. Nickersone,g, Herbert M. Saurof,g,h aCenter for Cell Analysis and Modeling, UConn Health, Farmington, CT, US bDepartment of Biomedical Informatics and Medical Education, University of Washington, Seattle, WA, US cIcahn Institute for Data Science and Genomic Technology, Icahn School of Medicine at Mount Sinai, New York, NY, US dDepartment of Genetics and Genomic Sciences, Icahn School of Medicine at Mount Sinai, New York, NY, US eAuckland Bioengineering Institute, University of Auckland, Auckland, NZ fDepartment of Bioengineering, University of Washington, Seattle, WA, US gThese authors contributed equally to this work hCorrespondence: [email protected] Abstract Although reproducibility is a core tenet of the scientific method, it remains challenging to re- produce many results. Surprisingly, this also holds true for computational results in domains such as systems biology where there have been extensive standardization efforts. For exam- ple, Tiwari et al. recently found that they could only repeat 50% of published simulation results in systems biology. Toward improving the reproducibility of computational systems research, we identified several resources that investigators can leverage to make their research more accessible, executable, and comprehensible by others. In particular, we identified sev- eral domain standards and curation services, as well as powerful approaches pioneered by the software engineering industry that we believe many investigators could adopt. Together, we believe these approaches could substantially enhance the reproducibility of systems biology research. In turn, we believe enhanced reproducibility would accelerate the development of more sophisticated models that could inform precision medicine and synthetic biology. -
Revised Glossary for AQA GCSE Biology Student Book
Biology Glossary amino acids small molecules from which proteins are A built abiotic factor physical or non-living conditions amylase a digestive enzyme (carbohydrase) that that affect the distribution of a population in an breaks down starch ecosystem, such as light, temperature, soil pH anaerobic respiration respiration without using absorption the process by which soluble products oxygen of digestion move into the blood from the small intestine antibacterial chemicals chemicals produced by plants as a defence mechanism; the amount abstinence method of contraception whereby the produced will increase if the plant is under attack couple refrains from intercourse, particularly when an egg might be in the oviduct antibiotic e.g. penicillin; medicines that work inside the body to kill bacterial pathogens accommodation ability of the eyes to change focus antibody protein normally present in the body acid rain rain water which is made more acidic by or produced in response to an antigen, which it pollutant gases neutralises, thus producing an immune response active site the place on an enzyme where the antimicrobial resistance (AMR) an increasing substrate molecule binds problem in the twenty-first century whereby active transport in active transport, cells use energy bacteria have evolved to develop resistance against to transport substances through cell membranes antibiotics due to their overuse against a concentration gradient antiretroviral drugs drugs used to treat HIV adaptation features that organisms have to help infections; they -
Andrea Deoudes, Kinetics: a Clock Reaction
A Kinetics Experiment The Rate of a Chemical Reaction: A Clock Reaction Andrea Deoudes February 2, 2010 Introduction: The rates of chemical reactions and the ability to control those rates are crucial aspects of life. Chemical kinetics is the study of the rates at which chemical reactions occur, the factors that affect the speed of reactions, and the mechanisms by which reactions proceed. The reaction rate depends on the reactants, the concentrations of the reactants, the temperature at which the reaction takes place, and any catalysts or inhibitors that affect the reaction. If a chemical reaction has a fast rate, a large portion of the molecules react to form products in a given time period. If a chemical reaction has a slow rate, a small portion of molecules react to form products in a given time period. This experiment studied the kinetics of a reaction between an iodide ion (I-1) and a -2 -1 -2 -2 peroxydisulfate ion (S2O8 ) in the first reaction: 2I + S2O8 I2 + 2SO4 . This is a relatively slow reaction. The reaction rate is dependent on the concentrations of the reactants, following -1 m -2 n the rate law: Rate = k[I ] [S2O8 ] . In order to study the kinetics of this reaction, or any reaction, there must be an experimental way to measure the concentration of at least one of the reactants or products as a function of time. -2 -2 -1 This was done in this experiment using a second reaction, 2S2O3 + I2 S4O6 + 2I , which occurred simultaneously with the reaction under investigation. Adding starch to the mixture -2 allowed the S2O3 of the second reaction to act as a built in “clock;” the mixture turned blue -2 -2 when all of the S2O3 had been consumed. -
Connectivity and Complex Systems: Learning from a Multi-Disciplinary Perspective
This is a repository copy of Connectivity and complex systems: learning from a multi-disciplinary perspective. White Rose Research Online URL for this paper: http://eprints.whiterose.ac.uk/141950/ Version: Published Version Article: Turnbull, L., Hütt, M-T., Ioannides, A.A. et al. (8 more authors) (2018) Connectivity and complex systems: learning from a multi-disciplinary perspective. Applied Network Science, 3 (11). ISSN 2364-8228 https://doi.org/10.1007/s41109-018-0067-2 Reuse This article is distributed under the terms of the Creative Commons Attribution (CC BY) licence. This licence allows you to distribute, remix, tweak, and build upon the work, even commercially, as long as you credit the authors for the original work. More information and the full terms of the licence here: https://creativecommons.org/licenses/ Takedown If you consider content in White Rose Research Online to be in breach of UK law, please notify us by emailing [email protected] including the URL of the record and the reason for the withdrawal request. [email protected] https://eprints.whiterose.ac.uk/ Turnbull et al. Applied Network Science (2018) 3:11 https://doi.org/10.1007/s41109-018-0067-2 Applied Network Science REVIEW Open Access Connectivity and complex systems: learning from a multi-disciplinary perspective Laura Turnbull1* , Marc-Thorsten Hütt2, Andreas A. Ioannides3, Stuart Kininmonth4,5, Ronald Poeppl6, Klement Tockner7,8,9, Louise J. Bracken1, Saskia Keesstra10, Lichan Liu3, Rens Masselink10 and Anthony J. Parsons11 * Correspondence: Laura.Turnbull@ durham.ac.uk Abstract 1Durham University, Durham, UK Full list of author information is In recent years, parallel developments in disparate disciplines have focused on what available at the end of the article has come to be termed connectivity; a concept used in understanding and describing complex systems. -
Chapter 10 – Chemical Reactions Notes
Chapter 8 – Chemical Reactions Notes Chemical Reactions: Chemical reactions are processes in which the atoms of one or more substances are rearranged to form different chemical compounds. How to tell if a chemical reaction has occurred (recap): Temperature changes that can’t be accounted for. o Exothermic reactions give off energy (as in fire). o Endothermic reactions absorb energy (as in a cold pack). Spontaneous color change. o This happens when things rust, when they rot, and when they burn. Appearance of a solid when two liquids are mixed. o This solid is called a precipitate. Formation of a gas / bubbling, as when vinegar and baking soda are mixed. Overall, the most important thing to remember is that a chemical reaction produces a whole new chemical compound. Just changing the way that something looks (breaking, melting, dissolving, etc) isn’t enough to qualify something as a chemical reaction! Balancing Equations Notes: Things to keep in mind when looking at the recipes for chemical reactions: 1) The stuff before the arrow is referred to as the “reactants” or “reagents”, and the stuff after the arrow is called the “products.” 2) The number of atoms of each element is the same on both sides of the arrow. Even though there may be different numbers of molecules, the number of atoms of each element needs to remain the same to obey the law of conservation of mass. 3) The numbers in front of the formulas tell you how many molecules or moles of each chemical are involved in the reaction. 4) Equations are nothing more than chemical recipes. -
Part III Project 2016/17 Professor Chris Hunter Physical Organic
Department of Chemistry: Part III Project 2016/17 Professor Chris Hunter Physical Organic Chemistry or Synthetic Supramolecular Chemistry EMAIL [email protected] Group web site http://www-hunter.ch.cam.ac.uk/ Contact details: I will be available to discuss projects on Monday 15th May (please email to make an appointment) The success of synthetic chemistry in the last century was built on the development of a set of design rules that allowed the quantitative prediction of reactivity and conformation based simply on chemical structure. The goal of our research is to establish a comparable set of rules that can be used for the design of non-covalent systems with equal reliability. Research projects are available in different areas and can be tailored to involve combinations of different techniques: organic synthesis; coordination chemistry; structural and thermodynamic characterisation of intermolecular complexes using NMR spectroscopy, mass spectrometry, X-ray crystallography; high-throughput physical organic chemistry; molecular design and molecular modelling. Physical Organic Chemistry: Quantitative Non-Covalent Chemistry Synthetic supramolecular systems are ideally suited for the systematic study and quantitative determination of the thermodynamic properties of non-covalent interactions. We are developing new experimental methods for quantifying the relative contributions of different factors that influence the behaviour of complex systems. By characterising the relationship between chemical structure and thermodynamic properties, we aim to develop rules of thumb (and software) for predicting the properties of molecular systems based on a quantitative fundamental understanding of non-covalent interactions. This project will involve some synthetic chemistry, but the focus will be on quantitative physical measurements using a variety of spectroscopic techniques and instrumentation as well as mathematical model building. -
Study Guide Medical Terminology by Thea Liza Batan About the Author
Study Guide Medical Terminology By Thea Liza Batan About the Author Thea Liza Batan earned a Master of Science in Nursing Administration in 2007 from Xavier University in Cincinnati, Ohio. She has worked as a staff nurse, nurse instructor, and level department head. She currently works as a simulation coordinator and a free- lance writer specializing in nursing and healthcare. All terms mentioned in this text that are known to be trademarks or service marks have been appropriately capitalized. Use of a term in this text shouldn’t be regarded as affecting the validity of any trademark or service mark. Copyright © 2017 by Penn Foster, Inc. All rights reserved. No part of the material protected by this copyright may be reproduced or utilized in any form or by any means, electronic or mechanical, including photocopying, recording, or by any information storage and retrieval system, without permission in writing from the copyright owner. Requests for permission to make copies of any part of the work should be mailed to Copyright Permissions, Penn Foster, 925 Oak Street, Scranton, Pennsylvania 18515. Printed in the United States of America CONTENTS INSTRUCTIONS 1 READING ASSIGNMENTS 3 LESSON 1: THE FUNDAMENTALS OF MEDICAL TERMINOLOGY 5 LESSON 2: DIAGNOSIS, INTERVENTION, AND HUMAN BODY TERMS 28 LESSON 3: MUSCULOSKELETAL, CIRCULATORY, AND RESPIRATORY SYSTEM TERMS 44 LESSON 4: DIGESTIVE, URINARY, AND REPRODUCTIVE SYSTEM TERMS 69 LESSON 5: INTEGUMENTARY, NERVOUS, AND ENDOCRINE S YSTEM TERMS 96 SELF-CHECK ANSWERS 134 © PENN FOSTER, INC. 2017 MEDICAL TERMINOLOGY PAGE III Contents INSTRUCTIONS INTRODUCTION Welcome to your course on medical terminology. You’re taking this course because you’re most likely interested in pursuing a health and science career, which entails proficiencyincommunicatingwithhealthcareprofessionalssuchasphysicians,nurses, or dentists. -
Chemistry - B.S
Chemistry - B.S. College of (Biochemistry Option) Arts and Sciences The Department of Chemistry offers the Bachelor of Science degree for students who Graduation Composition and Communication Requirement intend to become professional chemists or do graduate work in chemistry or a closely (GCCR) related discipline. There are three options in the B.S. program: a traditional track WRD 310 Writing in the Natural Sciences ............................................................. 3 covering all the major areas of chemistry, an option that emphasizes biochemistry and an option in materials chemistry. The Biochemistry and Traditional Options are Graduation Composition and Communication certified by the American Chemical Society. A Bachelor of Arts degree program is Requirement hours (GCCR) .................................................................... 3 offered as well for students who want greater flexibility in the selection of courses to perhaps pursue more diverse degree options, including dual and double majors. For College Requirements all majors CHE 109 and CHE 110 have been defined as equivalent to CHE 105. The I. Foreign Language (placement exam recommended) ................................... 0-14 Department also offers the Master of Science and the Doctor of Philosophy degree. II. Disciplinary Requirements a. Natural Science (completed by Major Requirements) 128 hours b. Social Science ......................................................................................... 3 Any student earning a Bachelor of Science (BS)