Gas Phase Acidity and Proton Affinity Studies of Organic
Total Page:16
File Type:pdf, Size:1020Kb
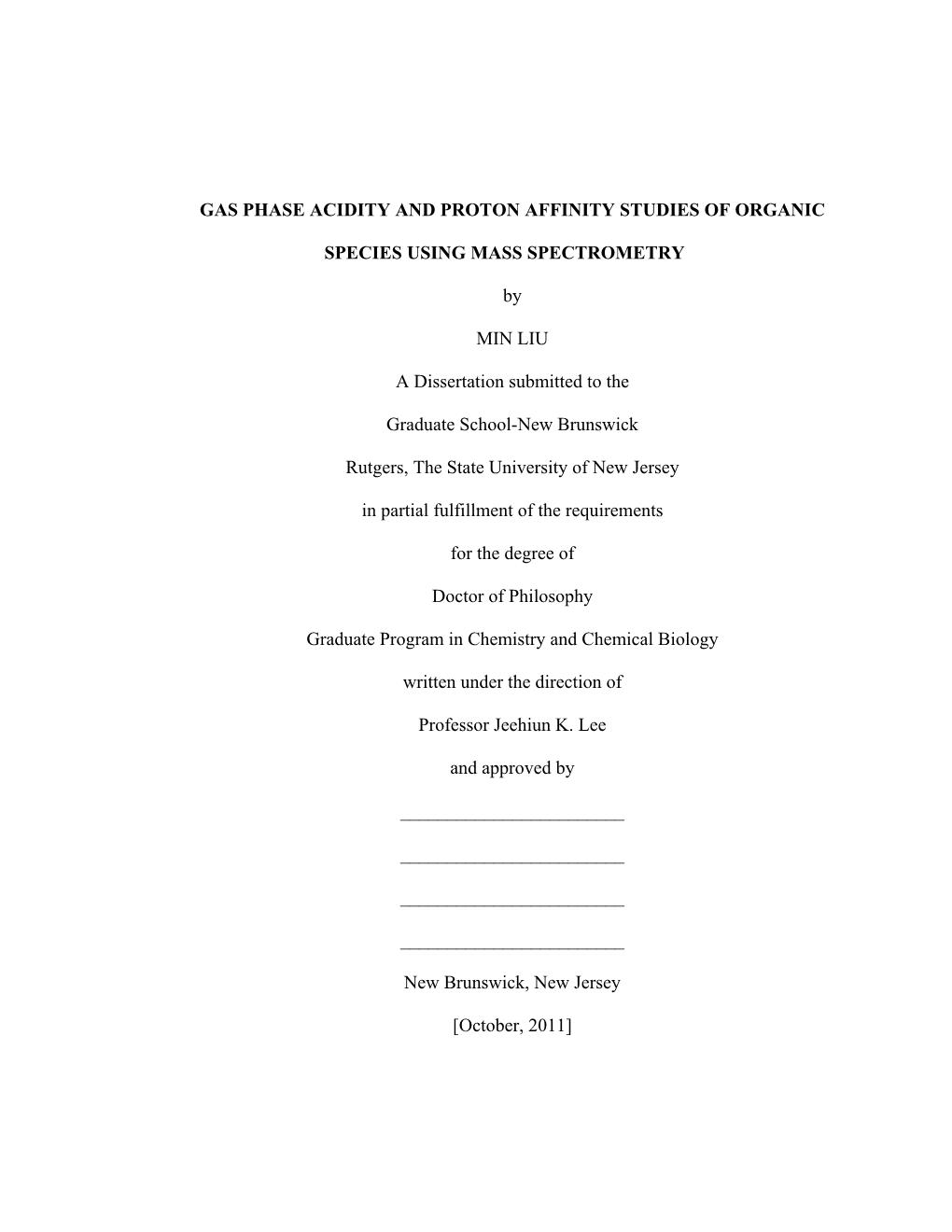
Load more
Recommended publications
-
Proton Affinity Changes Driving Unidirectional Proton Transport In
doi:10.1016/S0022-2836(03)00903-3 J. Mol. Biol. (2003) 332, 1183–1193 Proton Affinity Changes Driving Unidirectional Proton Transport in the Bacteriorhodopsin Photocycle Alexey Onufriev1, Alexander Smondyrev2 and Donald Bashford1* 1Department of Molecular Bacteriorhodopsin is the smallest autonomous light-driven proton pump. Biology, The Scripps Research Proposals as to how it achieves the directionality of its trans-membrane Institute, 10550 North Torrey proton transport fall into two categories: accessibility-switch models in Pines Road, La Jolla, CA 92037 which proton transfer pathways in different parts of the molecule are USA opened and closed during the photocycle, and affinity-switch models, which focus on changes in proton affinity of groups along the transport 2Schro¨dinger Inc., 120 West chain during the photocycle. Using newly available structural data, and Forty-Fifth Street, 32nd Floor adapting current methods of protein protonation-state prediction to the Tower 45, New York, NY non-equilibrium case, we have calculated the relative free energies of pro- 10036-4041, USA tonation microstates of groups on the transport chain during key confor- mational states of the photocycle. Proton flow is modeled using accessibility limitations that do not change during the photocycle. The results show that changes in affinity (microstate energy) calculable from the structural models are sufficient to drive unidirectional proton trans- port without invoking an accessibility switch. Modeling studies for the N state relative to late M suggest that small structural re-arrangements in the cytoplasmic side may be enough to produce the crucial affinity change of Asp96 during N that allows it to participate in the reprotonation of the Schiff base from the cytoplasmic side. -
Proton Affinity of SO3
View metadata, citation and similar papers at core.ac.uk brought to you by CORE provided by Elsevier - Publisher Connector Proton Affinity of SO3 Cynthia Ann Pommerening, Steven M. Bachrach, and Lee S. Sunderlin Department of Chemistry, Northern Illinois University, DeKalb, Illinois, USA ϩ Collision-induced dissociation (CID) of the radical cation H2SO4 gives the product pairs ϩ ϩ ϩ ϩ H2O SO3 and HO HSO3 with a 1:3 ratio that is essentially independent of collision energy. Statistical analysis of the two channels indicates that the proton affinity of HO is 3 Ϯ ϭ Ϯ 4 kJ/mol lower than that of SO3. This can be used to derive PA(SO3) 591 4 kJ/mol at 0 K and 596 Ϯ 4 kJ/mol at 298 K. Previously, Munson and Smith bracketed the proton affinity as PA(HBr) ϭ 584 kJ/mol Ͻ PA(SO ) Ͻ PA(CO) ϭ 594 kJ/mol. The threshold of 152 Ϯ 16 ϩ 3 kJ/mol for formation of H O ϩ SO indicates that the barrier to CID is small or nonexistent, 2 3 ϩ in contrast to the substantial barriers to decomposition for H3SO4 and H2SO4. (JAmSoc Mass Spectrom 1999, 10, 856–861) © 1999 American Society for Mass Spectrometry he development of extensive scales of proton this work provide an independent measurement of the ⌬ affinity (PA), gas basicity (GB), and acidity ( Ha) PA of SO3. values has provided a framework for the quanti- The gas-phase addition of H2OtoSO3 to form T ϭϪ⌬ tative understanding of ion properties. (PA H for sulfuric acid has a substantial barrier, as indicated by addition of a proton, GB ϭϪ⌬G for addition of a reaction rate measurements [4–6] and computational ⌬ ϭ⌬ proton, and Ha H for deprotonation.) The history results [7, 8]. -
Acid Dissociation Constant - Wikipedia, the Free Encyclopedia Page 1
Acid dissociation constant - Wikipedia, the free encyclopedia Page 1 Help us provide free content to the world by donating today ! Acid dissociation constant From Wikipedia, the free encyclopedia An acid dissociation constant (aka acidity constant, acid-ionization constant) is an equilibrium constant for the dissociation of an acid. It is denoted by Ka. For an equilibrium between a generic acid, HA, and − its conjugate base, A , The weak acid acetic acid donates a proton to water in an equilibrium reaction to give the acetate ion and − + HA A + H the hydronium ion. Key: Hydrogen is white, oxygen is red, carbon is gray. Lines are chemical bonds. K is defined, subject to certain conditions, as a where [HA], [A−] and [H+] are equilibrium concentrations of the reactants. The term acid dissociation constant is also used for pKa, which is equal to −log 10 Ka. The term pKb is used in relation to bases, though pKb has faded from modern use due to the easy relationship available between the strength of an acid and the strength of its conjugate base. Though discussions of this topic typically assume water as the solvent, particularly at introductory levels, the Brønsted–Lowry acid-base theory is versatile enough that acidic behavior can now be characterized even in non-aqueous solutions. The value of pK indicates the strength of an acid: the larger the value the weaker the acid. In aqueous a solution, simple acids are partially dissociated to an appreciable extent in in the pH range pK ± 2. The a actual extent of the dissociation can be calculated if the acid concentration and pH are known. -
Probing the Structure and Reactivity of Gaseous Ions a DISSERTATION
Probing the Structure and Reactivity of Gaseous Ions A DISSERTATION SUBMITTED TO THE FACULTY OF THE GRADUATE SCHOOL OF THE UNIVERSITY OF MINNESOTA BY Matthew Michael Meyer IN PARTIAL FULFILLMENT OF THE REQUIREMENTS FOR THE DEGREE OF DOCTOR OF PHILOSOPHY Professor Steven R. Kass Febuary 2010 © Matthew Meyer 2010 Acknowledgements I want to express my gratitude to my advisor Dr. Steven Kass for the opportunity to work with him during my time at Minnesota. I am grateful for his willingness to share not only his vast knowledge of chemistry, but his approach to addressing problems. I also would like to acknowledge professors, John Anthony and Mark Meier for their mentorship while I was at the University of Kentucky that led me to a career in chemistry. Due to the broad nature of the research contained herein I am grateful for the contributions of the many collaborators I had the opportunity to work with while at Minnesota. In particular, I would like to thank Professors Richard O’Hair, Steven Blanksby, and Mark Johnson for their wiliness to allow me to spend time in their labs. During my course of studies I have also had the opportunities to interacts with a variety of other scientist that have contributed greatly to my development and completing this document, including Dr. Dana Reed, Dr. Mark Juhasz, Dr. Erin Speetzen, Dr. Nicole Eyet and Mr. Kris Murphy. I am also grateful for the support of my brother and sister through out this process. Lastly, I want to expresses gratitude to my parents for their support in all my pursuits and encouraging my incessant asking why probably since I could talk. -
Gas-Phase Hydrogen/Deuterium Exchange As a Molecular Probe for the Interaction of Methanol and Protonated Peptides
View metadata, citation and similar papers at core.ac.uk brought to you by CORE provided by Elsevier - Publisher Connector Gas-Phase Hydrogen/Deuterium Exchange as a Molecular Probe for the Interaction of Methanol and Protonated Peptides Eric Gard, M. Kirk Green, Jennifer Bregar, and Carlito B. Lebrilla Departmentof Chemistry, University of California, Davis, California, USA The gas-phase hydrogen/deuterium (H/D) exchange kinetics of several protonated amino acids and dipeptides under a background pressure of CH,OD were determined in an external source Fourier transform mass spectrometer. H/D exchange reactions occur even when the gas-phase basicity of the compound is significantly larger (>20 kcal/mol) than methanol. In addition, greater deuterium incorporation is observed for compounds that have multiple sites of similar basicities. A mechanism is proposed that involves a structurally specific intermediate with extensive interaction between the protonated compound and methanol. (r Am Sot Mass Spectrom 1994,5, 623-631) he production of ionic gas-phase macro- [ 10, 11,18,19]. Investigations by Ausloos and L.ias [lOI molecules allows the possibility of studying these have shown that for protonated compounds H/D ex- T complex systems in the absence of solvent. Al- change reactions do not occur when the proton affinity though the gas and solvated phases are intrinsically of the neutral base is greater than the deuterated different, there is evidence to suggest that conforma- reagent by more than 20 kcal/mol. The correlation tion may be retained by the molecule even in the gas between basicity and reactivity has led many people to phase [l-5]. -
Gas-Phase Basicities of Polyfunctional Molecules. Part 2 : Saturated Basic Sites Guy Bouchoux, Jean-Yves Salpin
Gas-phase basicities of polyfunctional molecules. Part 2 : saturated basic sites Guy Bouchoux, Jean-Yves Salpin To cite this version: Guy Bouchoux, Jean-Yves Salpin. Gas-phase basicities of polyfunctional molecules. Part 2 : saturated basic sites. Mass Spectrometry Reviews, Wiley, 2012, 31 (3), pp.353-390. 10.1002/mas.20343. hal- 00760015 HAL Id: hal-00760015 https://hal.archives-ouvertes.fr/hal-00760015 Submitted on 5 Oct 2018 HAL is a multi-disciplinary open access L’archive ouverte pluridisciplinaire HAL, est archive for the deposit and dissemination of sci- destinée au dépôt et à la diffusion de documents entific research documents, whether they are pub- scientifiques de niveau recherche, publiés ou non, lished or not. The documents may come from émanant des établissements d’enseignement et de teaching and research institutions in France or recherche français ou étrangers, des laboratoires abroad, or from public or private research centers. publics ou privés. BOUCHOUX AND SALPIN POLYFUNCTIONAL MOLECULES WITH SATURATED BASIC SITES Gas-phase basicities of polyfunctional molecules. Part 2: saturated basic sites Guy Bouchoux1,2* and Jean-Yves Salpin3,4 (1) Ecole Polytechnique - Laboratoire des Mécanismes Réactionnels (DCMR) - Département de Chimie - 91120 Palaiseau. France. (2) CNRS – UMR 7651 (3) Université d'Evry Val d'Essonne - Laboratoire Analyse et Modélisation pour la Biologie et l'Environnement (LAMBE) – Bâtiment Maupertuis – Bd F. Mitterrand - 91025 Evry. France (4) CNRS – UMR 8587 * Correspondence to: Guy Bouchoux. Laboratoire des Mécanismes Réactionnels. Département de Chimie. Ecole Polytechnique. 91120 Palaiseau. France. E-mail address: [email protected] Telephone: (33) 1 69 33 48 42 FAX: (33) 1 69 33 48 03 -1- BOUCHOUX AND SALPIN POLYFUNCTIONAL MOLECULES WITH SATURATED BASIC SITES Table of contents I. -
Acid-Base Chemistry Gas-Phase Acid-Base Reactions the Most
5.03, Inorganic Chemistry Prof. Daniel G. Nocera Lecture 6 Apr 11: Acid-Base Chemistry Gas-Phase Acid-Base Reactions The most straightforward acid-base reaction occurs by attack of H+ on an atom or + molecule (B) in the gas phase. Consider the attack of H on H2 to produce the + simplest polyatomic molecule, H3 (which has been detected by mass spectrometry in electrical discharges of H2 gas). H+ attacks the HOMO of the base B for any protonation in the gas phase. For the example above, the bonding situation is described as follows: 1 + The difference in the bond dissociation energies of H2 and H3 gives the energy for + H association to H2, + + + –1 H3 → 2H + H ∆H1 = BDE(H3 ) = 203 kcal mol –1 H2 → 2H ∆H2 = BDE(H2) = 103 kcal mol Thus the energy for protonation is + + 2H + H → H3 H2 → 2H + + –1 H2 + H → H3 ∆Hassoc = ∆H2 – ∆H1 = –100 kcal mol and therefore the protonation of H2 is comparable to its bond strength. The proton affinity (PA) is the energy released upon attack of H+ on a species B in the gas phase, + + B + H → BH PA = –∆Hassoc by convention, a positive value is exothermic Can divide the protonation reaction in to two hypothetical reactions + + B + H → B + H ∆H1 = IE(B) – IE(H) + + + B + H → BH ∆H2 = –BDE(BH ) + + + B + H → BH ∆Hassoc = IE(B) – IE(H) – BDE(BH ) PA = IE(H) – IE(B) + BDE(BH+) PA = 13.598 eV – IE(B) + BDE(BH+) Protonation of B is therefore favored for small IE(B) … i.e., for small ionization energies of electrons in HOMO (or in other terms, electrons in higher energy HOMO are more easily attacked by the proton) and large bond dissociation energies. -
Preparation of an Ion with the Highest Calculated Proton Affinity: Ortho- Diethynylbenzene Dianion
University of Wollongong Research Online Faculty of Science, Medicine and Health - Papers: part A Faculty of Science, Medicine and Health 1-1-2016 Preparation of an ion with the highest calculated proton affinity: ortho- diethynylbenzene dianion Berwyck L. J Poad University of Wollongong, [email protected] Nicholas D. Reed University of Wollongong, [email protected] Christopher Hansen University of Wollongong, [email protected] Adam J. Trevitt University of Wollongong, [email protected] Stephen J. Blanksby Queensland University of Technology, [email protected] See next page for additional authors Follow this and additional works at: https://ro.uow.edu.au/smhpapers Part of the Medicine and Health Sciences Commons, and the Social and Behavioral Sciences Commons Recommended Citation Poad, Berwyck L. J; Reed, Nicholas D.; Hansen, Christopher; Trevitt, Adam J.; Blanksby, Stephen J.; Mackay, Emily G.; Sherburn, Michael S.; Chan, Bun; and Radom, Leo, "Preparation of an ion with the highest calculated proton affinity: ortho-diethynylbenzene dianion" (2016). Faculty of Science, Medicine and Health - Papers: part A. 4094. https://ro.uow.edu.au/smhpapers/4094 Research Online is the open access institutional repository for the University of Wollongong. For further information contact the UOW Library: [email protected] Preparation of an ion with the highest calculated proton affinity: ortho- diethynylbenzene dianion Abstract Owing to the increased proton affinity that results from additional negative charges, multiply-charged anions have been proposed as one route to prepare and access a range of new and powerful "superbases". Paradoxically, while the additional electrons in polyanions increase basicity they serve to diminish the electron binding energy and thus, it had been thought, hinder experimental synthesis. -
Acids, Bases and Solution Equilibria
Acids, bases and solution equilibria A four lecture course for the 1st year Jose M. Goicoechea http://course.chem.ox.ac.uk/acids-bases-and- solutions-equilibria-year-1-2014.aspx http://goicoechea.chem.ox.ac.uk/teaching.html Acid-base reactions + + NH3 + H3O NH4 + H2O + - H2O + HI H3O + I - 2- + HSO4 + H2O SO4 + H3O NH3 + BF3 NH3:BF3 C5H5N + I2 C5H5N:I2 Species highlighted act as acids Redox reactions A redox reaction is a reaction in which there is a change in oxidation state Fe3+(aq) + Cr2+(aq) Fe2+(aq) + Cr3+(aq) + 2+ Zn(s) + 2H3O Zn + H2(g) 2PCl3 + O2 2OPCl3 Ca(s) + H2 CaH2(s) + - O2 + Pt + 3F2 [O2] [PtF6] One or two electrons are transferred entirely Species highlighted act as oxidants Definitions of Acid/Base Arrhenius/Ostwald Brønsted/Lowry Lux/Flood ‘Solvent system’ Lewis Usanovich Arrhenius/Ostwald Acids and bases dissociate in H2O, + + releasing H (H3O ) and OH-. Arrhenius Ostwald + - H2O H (aq) + OH (aq) + H (aq) is an acid - OH (aq) is a base Brønsted/Lowry Proton theory retained but the definition is now independent of solvent Brønsted Lowry An acid is a proton donor and a base is a proton acceptor. + - Other solvents are also NH4 + NH2 2NH3 capable of self-ionisation + - Proton HCl + NH3 [NH ] Cl transferred from 4 acid to base Donor acid Acceptor base Brønsted/Lowry Every acid has a conjugate base and every base has a conjugate acid HA + B- A- + HB The conjugate base of a weak acid is a strong base, and the conjugate base of a strong acid is a weak base. -
Acid–Base and Donor– Acceptor Chemistry
Chapter 6 Acid–Base and Donor– Acceptor Chemistry 6.1 Acid–Base Models as Organizing Concepts A long-standing chemical objective is to organize reactions by using models to account for trends and gain insight into what properties of reactants are prerequisites for chemical change. Analyzing trends among similar reactions permits discovery of structure–function relationships (for example, how do molecular geometry and electronic structure influence reactivity?) and guides the design of molecules for practical use. Classifying substances as acids and bases has been important since ancient times; alchemists used neutralization—the ubiquitous reaction of an acid and base to form salt and water—to compile observations about different substances that engaged in similar reactions. Without modern structural analysis tools, such as X-ray crystallography and NMR spectroscopy, alchemists used their senses: they observed the tastes of acids (sour) and bases (bitter) and color changes of indicators. Many acid–base definitions have been devised, but only a few have been widely adopted. This chapter discusses the major acid–base models and their application in inorganic chemistry. After a historical introduction (Section 6.1.1), the models are presented in the rough order of their development. Among these are the ones attributed to Arrhenius (Section 6.2), Brønsted–Lowry (Section 6.3), and Lewis (Section 6.4). These sections emphasize the challenges associated with quantifying acidity and basicity, and rela- tionships between acid/base strength and molecular structure. The 1960s application of molecular orbitals (i.e., HOMO/LUMO interactions) to frame Lewis acid–base reactions (Section 6.4.1) permeates inorganic chemistry and dramatically expands the perspective on what constitutes an acid–base reaction. -
Ortho-Diethynylbenzene Dianion† Cite This: Chem
Chemical Science View Article Online EDGE ARTICLE View Journal | View Issue Preparation of an ion with the highest calculated proton affinity: ortho-diethynylbenzene dianion† Cite this: Chem. Sci.,2016,7,6245 Berwyck L. J. Poad,ab Nicholas D. Reed,b Christopher S. Hansen,b Adam J. Trevitt,b Stephen J. Blanksby,a Emily G. Mackay,c Michael S. Sherburn,c Bun Chan‡d and Leo Radomd Owing to the increased proton affinity that results from additional negative charges, multiply-charged anions have been proposed as one route to prepare and access a range of new and powerful “superbases”. Paradoxically, while the additional electrons in polyanions increase basicity they serve to diminish the electron binding energy and thus, it had been thought, hinder experimental synthesis. We report the synthesis and isolation of the ortho-diethynylbenzene dianion (ortho-DEB2À) and present observations of this novel species undergoing gas-phase proton-abstraction reactions. Using a theoretical model based on Marcus–Hush theory, we attribute the stability of ortho-DEB2À to the Received 20th April 2016 presence of a barrier that prevents spontaneous electron detachment. The proton affinity of 1843 kJ Creative Commons Attribution 3.0 Unported Licence. Accepted 17th June 2016 molÀ1 calculated for this dianion superbase using high-level quantum chemistry calculations significantly DOI: 10.1039/c6sc01726f exceeds that of the lithium monoxide anion, the most basic system previously prepared. The ortho- www.rsc.org/chemicalscience diethynylbenzene dianion is therefore the -
Inorganic Chemistry
Inorganic Chemistry Acids, Bases and Non-Aqueous Solvents Prof. K N Upadhya 326 SFS Flats Ashok Vihar Phase IV Delhi -110052 CONTENTS Introduction Arrhenius Concept Bronsted-Lowry Concept of Acids and Bases Solvent-System Concept Aprotic Acids and Bases Lux-Flood Acid-Base Concept Strength of Bronsted Acids and Bases Relative Strengths of Acids and Bases Solvent Levelling and Discrimination in Water Amphoterism Trends in Acid Strength of various Bronsted Acids Acid Strength and Molecular Structure Strength of Mononuclear Oxoacids- Pauling’s Rules Lewis Acids and Bases Hard and Soft Acids and Bases Autoionization of Solvents Reactions in Non-Aqueous Solvents Discrimination in Non-Aqueous Solvents Keywords Arrhenius theory, Bronsted-Lowry theory, Solvent-system concept 1 Introduction The terms ‘acids’ and ‘bases’ have been defined in many ways. According to Arrhenius, probably the oldest, acids, and bases are the sources of H+ and OH- ions respectively. A somewhat broader but closely related definition (Bronsted – Lowry) is that an acid is a substance that supplies protons and a base is proton acceptor. Thus in water an acid increases the concentration of hydrated proton (H3O+) and a base lowers it or increases the concentration of OH-. In addition to Bronsted-Lowry concept there are others like solvent- solvent definition and the Lux and Flood definition but each one has its own limitations. One of the most general and useful of all definitions in reference to complex formations was due to G. N. Lewis. Lewis defined acid-base in terms of electron pair donor-acceptor capability. This definition includes Bronsted- Lowry definition as a special case.