Water Resources and Dynamics of the Troodos Igneous Aquifer-System, Cyprus - Balanced Groundwater Modelling
Total Page:16
File Type:pdf, Size:1020Kb
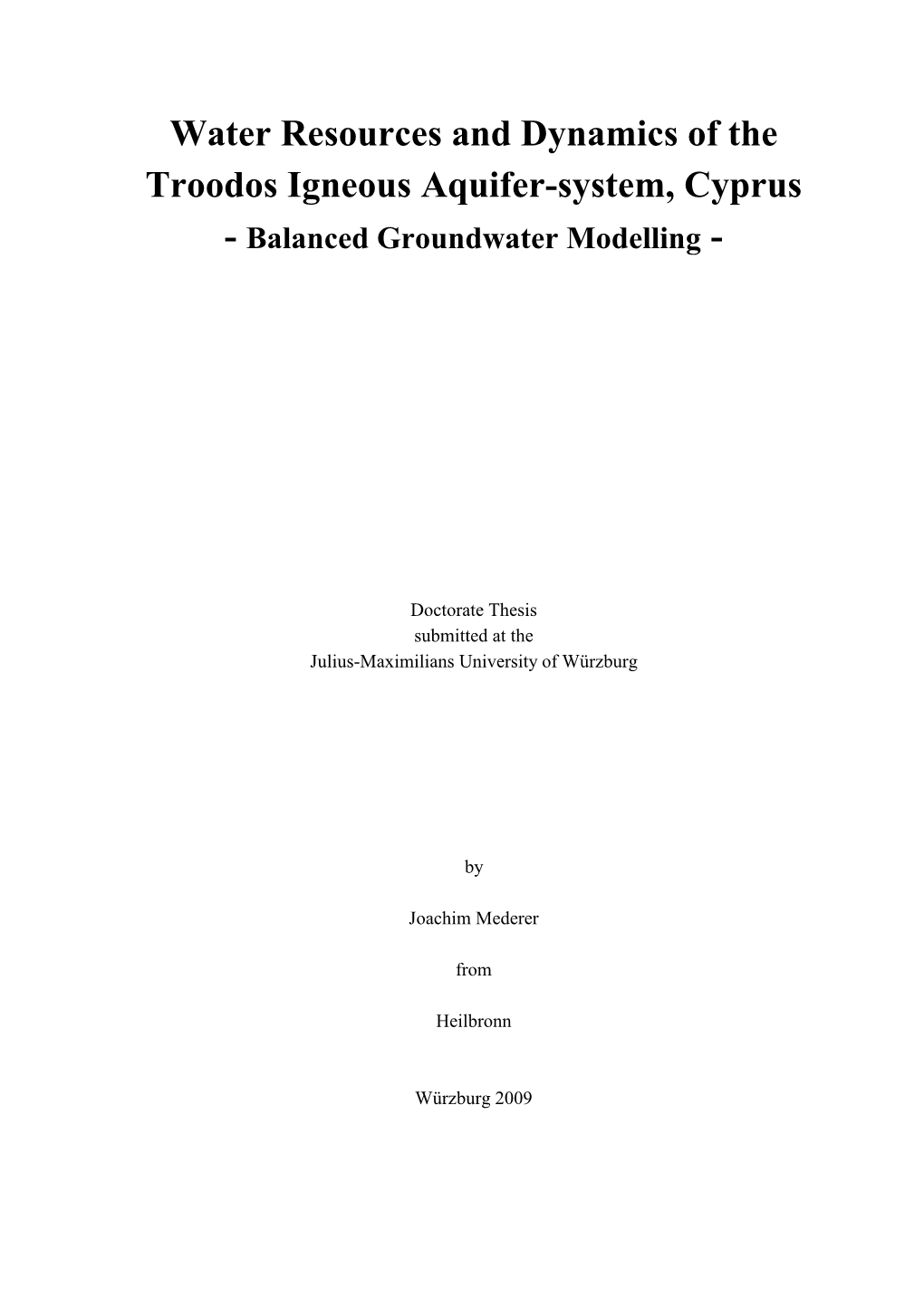
Load more
Recommended publications
-
CYPRUS Cyprus in Your Heart
CYPRUS Cyprus in your Heart Life is the Journey That You Make It It is often said that life is not only what you are given, but what you make of it. In the beautiful Mediterranean island of Cyprus, its warm inhabitants have truly taken the motto to heart. Whether it’s an elderly man who basks under the shade of a leafy lemon tree passionately playing a game of backgammon with his best friend in the village square, or a mother who busies herself making a range of homemade delicacies for the entire family to enjoy, passion and lust for life are experienced at every turn. And when glimpsing around a hidden corner, you can always expect the unexpected. Colourful orange groves surround stunning ancient ruins, rugged cliffs embrace idyllic calm turquoise waters, and shady pine covered mountains are brought to life with clusters of stone built villages begging to be explored. Amidst the wide diversity of cultural and natural heritage is a burgeoning cosmopolitan life boasting towns where glamorous restaurants sit side by side trendy boutiques, as winding old streets dotted with quaint taverns give way to contemporary galleries or artistic cafes. Sit down to take in all the splendour and you’ll be made to feel right at home as the locals warmly entice you to join their world where every visitor is made to feel like one of their own. 2 Beachside Splendour Meets Countryside Bliss Lovers of the Mediterranean often flock to the island of Aphrodite to catch their breath in a place where time stands still amidst the beauty of nature. -
About Limassol
ABOUT LIMASSOL The district of Limassol which covers the south part of Cyprus, borders with the district of Larnaca in the east, with the district of Paphos in the west, as well as with the capital Nicosia in the north. The area of Limassol is 1,393 km² i.e. 15% of the whole area of Cyprus. About 80 km of Limassol is washed by the sea. Combining its roles as the second largest city, the island's main port, the centre of the wine industry and a bustling holiday resort, Lemesos emerges as a spirited and cosmopolitan seaside town. Limassol is a lively town largely due to the character of Lemesolians, a fun- loving lot. No wonder it holds the island's two top festivals, the pre-lenten Carnival with fancy dress balls, parades and festivities and the Wine Festival in September, a wine extravaganza where wine flows freely for everyone to enjoy, courtesy of the local wineries. Limassol emerged out of the two most important ancient city-kingdoms, Amathous, to the east of the town, and Kourion to the west, both of which are being extensively excavated. The magnificent setting of the ancient Kourion Theater is used for summer concerts and theatrical productions. In the middle Ages, Limassol hosted the marriage of Richard the Lionheart with Berengaria of Navarre from whom he crowned Queen of England. Thereafter the Crusaders made their headquarters at the Square keep west of the city, known as Kolossi Medieval Castle, where they fostered the making of wines, particularly the sweet dessert wine “Commandaria” – the oldest named wine in the world. -
13 Days in Cyprus
13 days in Cyprus Contact us | turipo.com | [email protected] 13 days in Cyprus 13 days in Cyprus: Larnaca, Aya Napa, Trodos mountain, Phapos and more... Contact us | turipo.com | [email protected] Day 1 - Arrive in Larnaca Contact us | turipo.com | [email protected] Day 1 - Arrive in Larnaca 1. Larnaca International Airport Duration ~ 2 Hours Larnaca, Cyprus Monday: Open 24 hours Tuesday: Open 24 hours Wednesday: Open 24 hours Thursday: Open 24 hours Friday: Open 24 hours Saturday: Open 24 hours Sunday: Open 24 hours Telephone: +357 77 778833 Website: www.hermesairports.com Rating: 4.2 2. Ayia Napa Ayia Napa, Cyprus 3. Ayia Napa Harbour 1st October, Cyprus Rating: 4.6 4. Konnos Beach Konnos Beach, Cyprus Rating: 4.7 Contact us | turipo.com | [email protected] Day 2 - Aya Napa Contact us | turipo.com | [email protected] Day 2 - Aya Napa Wednesday: Open 24 hours 1. Ayia Napa Thursday: Open 24 hours Friday: Open 24 hours Ayia Napa, Cyprus Saturday: Open 24 hours Sunday: Open 24 hours 2. WaterWorld Themed Waterpark Ayia Napa Rating: 4.6 18, Ayia Thekla Road, Agia Napa (Ayia Napa) 5345, Cyprus 5. The Blue Lagoon Telephone: +357 23 724444 Website: waterworldwaterpark.com Ayia Napa, Cyprus Rating: 4.4 Monday: Open 24 hours Tuesday: Open 24 hours 3. Cape Greco National Forest Park Wednesday: Open 24 hours E307, Ayia Napa, Cyprus Thursday: Open 24 hours Friday: Open 24 hours Saturday: Open 24 hours Monday: Open 24 hours Sunday: Open 24 hours Tuesday: Open 24 hours Wednesday: Open 24 hours Rating: 4.7 Thursday: Open 24 hours Friday: Open 24 hours WIKIPEDIA Saturday: Open 24 hours Blue Lagoon may refer to: Sunday: Open 24 hours Telephone: +357 22 805511 Rating: 4.7 4. -
16 >19 of November 2017
Field Study Tour 16 >19 of November 2017 Road Map 1 About the organizers RURENER is a European network of rural stakeholders committed to the energy transition. Our vision is to build a European Union self-conscious of the potentials of its territories, and especially its rural territories, to realize the energy transition. By addressing energy challenges, we promote a transversal approach of rural development. Through our members’ experiences, we advocate for the crucial role of rural territories in achieving the energy transition in Europe, and we unite rural territories’ voice to make it heard and acknowledged in Brussels. The network is a privileged place to share good practices, transfer knowledge and build cooperation projects with European partners. RURENER members are communities and other RURENER territorial entities, networks, organizations and individuals, from all over Europe. This diversity rurener.eu reflects our vision of a plural rural Europe. PESAP - The Regional Union of former residents Associations of Pitsilia is the coordinating body of former residents Associations from 30 Communities in the region of Pitsilia, in both the Nicosia and the District of Limassol. The Pitsilia area is a mountainous area, the largest of Troodos system and the communities are all located at an altitude of over 800 meters. The isolated area is in decline demographically and economically and lacks a good road network connecting the area to the main urban areas. It is also lacking in terms of health, education and social services. Individual associations of former residents communities in the Pitsilia region decided to join forces and together with other local organizations to join forces to rejuvenate the region. -
Název Prezentace
10. Tourist attractions in the Asia Předmět: The Tourist Attractions in the Czech Republic and in the World Geography of Cyprus Cyprus is an island country in the Eastern Mediterranean and the third largest and third most populous island in the Mediterranean. Cyprus is located south of Turkey, west of Syria and Lebanon, northwest of Israel, north of Egypt, and southeast of Greece. A very narrow band of mountains (the Kyrenia) slices across the northern edge of Cyprus. That low range of mountains reaches a maximum of 1,024 m in elevation. In the south and western portions of the island the Troodos Mountains dominate. The highest point on Cyprus, located in the center of the Troodos range, is Mount Olympus at 1,952 m. Rivers are seasonal and only flow after heavy rain, and under those conditions the Pedieos is the longest river in Cyprus. It rises in the Troodos Mountains, flowing northeast through the capital city of Nicosia. It then steers east, meeting the sea at Famagusta Bay. The river has a total length of approximately 100 km. Cyprus has over 100 dams and reservoirs, and all are the island's principal source of water for both agricultural and domestic use. The main tourist attractions in Cyprus There's no shortage of ancient sites in Cyprus but Kourion is the pick of the bunch. Romantically situated across a coastal cliff with tumbling views of the countryside and Mediterranean below, it's a magical place. The entire site is vast, but the most famous section is the theater and the House of Eustolios, which holds a clutch of fine, well-preserved mosaics. -
Port Cities As Central Places in Early Roman Cyprus
land Article Transforming Culture on an Insula Portunalis: Port Cities as Central Places in Early Roman Cyprus Jody Michael Gordon Department of Humanities and Social Sciences, Wentworth Institute of Technology, Boston, MA 02115, USA; [email protected] Received: 15 November 2018; Accepted: 6 December 2018; Published: 9 December 2018 Abstract: During the Early Roman period in the Mediterranean (ca. 30 BC–330 AD), the key central places that distinguished socio-political landscapes were towns. These urban centers functioned as economic and administrative focal points that were controlled by local elites who oversaw wealth redistribution and maintained a dialectical relationship with Rome that mutually benefitted both parties. Yet, beyond providing such rudimentary observations, central place theory has recently been revised to examine how local factors, such as a place’s long-term geography and history, intersect with globalizing ones to transform settlement hierarchies as well as economic, political, and cultural landscapes. This article’s goal is to explore such intersections through a study of how port towns functioned as central places that connected globalized imperial networks to localized provincial ones within island contexts. It examines a range of material culture including, ceramics, architecture, prestige goods, and coinage from ports in Early Roman Cyprus in order to investigate how the island’s integration into Roman networks created central places that altered existing settlement types, hierarchies, and thus, local identities. Overall, this study shows how the reanalysis of central places within their unique geohistorical contexts can shed new light on both regional and state-level processes of cultural change. Keywords: Cyprus; Roman archaeology; Roman imperialism; island and coastal archaeology; identity; urbanism; central place theory; connectivity; maritime cultural landscapes 1. -
Troödos Mountains
©Lonely Planet Publications Pty Ltd Troödos Mountains Includes ¨ Why Go? Troödos ........................ 82 Home to Mt Olympus (1952m), the island’s highest peak, Platres ..........................84 this stunning mountain range provides visitors with a for- Omodos & ested flip side to the coastal resorts and big-city clamour. the Krasohoria ............. 85 Overlooking the valleys of Lemesos (Limassol), Larnaka and Pedoulas ...................... 87 the greater Mesaoria plain, this region covers over 90 sq km and is a protected natural park which safeguards its wild- Kalopanayiotis .............88 life, ecology and geology. In winter, skiers and snowboarders Kykkos populate the ski resorts of the northern slopes, while at oth- Monastery & Around ...89 er times of the year the park is ideal for camping, picnicking, Treis Elies ..................... 91 hiking, cycling and birdwatching. Pitsylia ......................... 93 In addition to the natural beauty of the landscape, the Agros ............................94 Troödos Mountains are home to a variety of postcard-pretty villages with cobbled streets, terraced slopes and vernacular architecture. The region’s peaks and valleys also hide some of the island’s most important medieval frescoed churches, Best Places to along with unexpected monasteries, museums and some of Eat the Republic’s finest wineries. ¨ Mylos Restaurant (p93) ¨ Mimi’s Restaurant (p85) When to Go ¨ Elyssia (p88) ¨ From January to April, you can experience the wintery ¨ Stou Kir Yianni (p86) pleasures of skiing and snowboarding the slopes by day, and enjoying a hearty tavern meal by night. ¨ Loutraki (p89) ¨ April to September is ideal for wine tasting; explore some of the superb wineries hidden among the sprawling vineyards Best Churches & and steep, breezy valleys. -
Walks in the Troodos Mountains of Cyprus Introduction by Chris Thompson 2003
Walks in the Troodos Mountains of Cyprus Introduction by Chris Thompson 2003 I have just experienced a wonderful walking holiday based in the resort of Platres (1200 metres / 3937 feet) which is situated in the Troodos (1920 metres / 6299 feet) mountain range on the fringe of the forests' southern slopes. The forests offer superb walking mostly along loose surface road tracks (disused mule trails or the old pre-tarmac road routes) with a comfortable mixture of open viewpoints and shade provided by the pine forests through which they have been cut. The local tourist authorities have provided a leaflet, which lists 11 walks in the area. It is not possible to obtain walking maps in Cyprus (or UK) and the tourist office maps have no detail. As there are no meaningful instructions provided in the leaflet, you could experience difficulty following some of the walks. I completed all the walks and found some path forks and junctions that were not sign-posted. If you make the wrong choice you could walk a considerable distance before you realise this, which tends to spoil the enjoyment! I decided to write this guide to assist fellow walkers - I hope you enjoy using it. I have included sufficient details for each walk, enabling you to approach them with complete confidence. An introduction to each walk offers simple advice and ideas for your consideration. I have listed the overall times and distances plus "time on route" between instructions, with totals in brackets. It is extremely difficult to offer an accurate time because personal walking speed, fitness and terrain will have considerable impact on timing. -
Authentic Cyprus Guide.Pdf
introShort This guidebook has been designed to provide visitors with an extensive insight into the delightful world of rural Cyprus. This is a world apart from the beaches and tourist hotspots. Here, timeless villages, tiny remote painted churches, stunning scenery, forested mountain trails and a way of life that has hardly changed over the past centuries, are just waiting to be discovered. The first part of this book provides general information on rural Cyprus, its history, traditions, cultures, flora and fauna, places of interest and more. The second half of the book, details 15 recommended driving excursions. All of the routes can be accomplished easily within a day’s drive in a regular car, yet all have something different to offer. The routes highlight points-of-interest along the way and all start and finish on one of the main roads. These routes are also ideal for organised group tours with small buses. The routes include places to stop for walking, cycling, bird watching, fresh-water fishing or to simply explore the countryside and charming villages. All that’s needed is a good road map, a sunhat, plenty of water, comfortable walking shoes and a spirit of adventure. NOTE: The spellings of all place-names conform to those indicated on the road signs. However, in some cases, these may vary from those shown on your road map. Contents Useful Information 3 Welcome to Rural Cyprus 4 Natural Environment 8 Cultural Heritage 12 Rural Crafts and Skills 18 Food and Wine 22 Rural Accommodation 28 Countryside Activities 32 Religious and Local -
Cheers Along! Wine Is Not a New Story for Cyprus
route5 Κoumandaria cheers along! Wine is not a new story for Cyprus. Recent archaeological excavations which have been undertaken on the island have confi rmed the thinking that this small tranche of earth has been producing wine for almost 5000 years. The discoveries testify that Cyprus may well be the cradle of wine development in the entire Mediterranean basin, from Greece, to Italy and France. This historic panorama of continuous wine history that the island possesses is just one Come -tour, taste of the reasons that make a trip to the wine villages such a fascinating prospect. A second and enjoy! important reason is the wines of today -fi nding and getting to know our regional wineries, which are mostly small and enchanting. Remember, though, it is important always to make contact fi rst to arrange your visit. The third and best reason is the wine you will sample during your journeys along the “Wine Routes” of Cyprus. From the traditional indigenous varieties of Mavro (for red and rosé wines) and the white grape Xynisteri, plus the globally unique Koumandaria to well - known global varieties, such as Chardonnay, Cabernet Sauvignon and Shiraz. Let’s take a wine walk. The wine is waiting for us! View from Monagri village 3 route Koumandaria 5 Lemesos, Kolossi, Erimi, Alassa, Agios Georgios Silikou, Doros, Laneia, Trimiklini, Agios Mamas, Kapileio, Zoopigi, Kalo Chorio, Agios Pavlos, Agios Konstantinos, Louvaras, Gerasa, Apsiou, Paramytha, Palodeia, Agia Fyla This route, travelling north from Lemesos, unites modern day vine growing and wine making with antiquity. Wines like those you will fi nd have been made right here for many centuries. -
Georgiou, Pauline. 2019. 'Staged Nostalgia': Negotiating Identity Through Encounters with the Landscapes of Conflict in Cypr
Georgiou, Pauline. 2019. ‘Staged Nostalgia’: Negotiating Identity through Encounters with the Landscapes of Conflict in Cyprus. Doctoral thesis, Goldsmiths, University of London [Thesis] https://research.gold.ac.uk/id/eprint/26977/ The version presented here may differ from the published, performed or presented work. Please go to the persistent GRO record above for more information. If you believe that any material held in the repository infringes copyright law, please contact the Repository Team at Goldsmiths, University of London via the following email address: [email protected]. The item will be removed from the repository while any claim is being investigated. For more information, please contact the GRO team: [email protected] ‘Staged Nostalgia’: Negotiating Identity through Encounters with the Landscapes of Conflict in Cyprus Pauline Georgiou For the degree of Doctor of Philosophy Department of Anthropology Goldsmiths, University of London 1 I …………………………………………….. hereby declare that this thesis and the work presented in it is entirely my own. Where I have consulted the work of others, this is always clearly stated. Signed: ______________________ Date: 2 ABSTRACT The thesis is an ethnography of modern day Greek Cypriot society and the struggle to establish identity and belonging through encounters with the landscape. It contributes to the anthropological literature on Cyprus and on wider literature on heritage, tourism and borders. The historical conflict that has left Cyprus divided for 44 years becomes the basis for an identity crisis which is then fuelled by internal conflicts based on perceptual dichotomies and divisions. Collectiveness is achieved through socializing mechanisms and recitals such as the proposed ‘staged nostalgia’ which involves the public and collective performance of patriotism, mourning, victimization and nostalgia. -
Development of Sustainability Indices for the Wider Area of Troodos in Cyprus
Island Sustainability 65 Development of sustainability indices for the wider area of Troodos in Cyprus A. Zorpas1, S. Malamis2, G. Lambrou2, E. Katsou2, M. Loizidou2 & I. Voukkali1 1Institute of Environmental Technology and Sustainable Development, Cyprus 2National Technical University of Athens, Department of Chemical Engineering, Laboratory of Environmental Science, Greece Abstract The concept of sustainability gathers together the various elements contributing to a human life support system on Earth and follows the seminal approach established by the Brundtl and report on sustainable development. Traditionally, sustainability is associated with criteria such as efficiency or equity from an economic, social and environmental viewpoint and deals with intra-generational and intergenerational issues. Indicators are considered of crucial importance for the measurement of “sustainability” in local contexts as well as for national and international policies, as they allow communicating, discussing and taking decisions on complex facts and trends, using relatively few data. The paper describes the development of sustainable indices for the wider area of Troodos in Cyprus which include 100 villages (that belong to two districts: Nicosia and Limassol), according to five criteria, which are considered as essential components of sustainable indices for the Troodos’ Mountains management. These criteria are: (i) society, (ii) cultural, (iii) economical and tourist (iv) geologic and hydrology (v) natural environment. The questionnaire’s main targets