How Control and Reasoning Processes Contribute to Adult Mentalizing
Total Page:16
File Type:pdf, Size:1020Kb
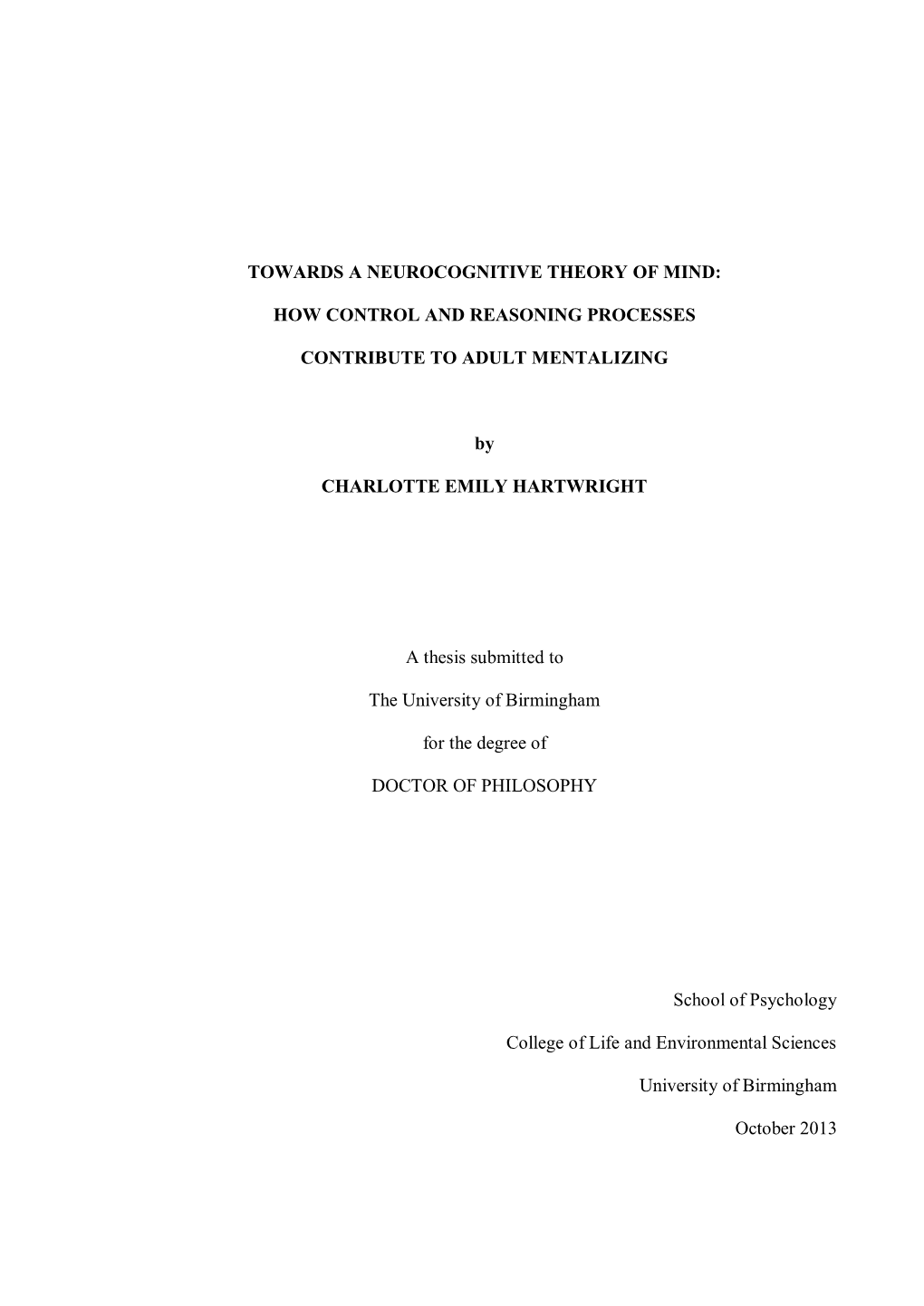
Load more
Recommended publications
-
The Neurological Asymmetry of Self-Face Recognition
S S symmetry Review The Neurological Asymmetry of Self-Face Recognition Aleksandra Janowska, Brianna Balugas, Matthew Pardillo, Victoria Mistretta, Katherine Chavarria, Janet Brenya, Taylor Shelansky, Vanessa Martinez, Kitty Pagano, Nathira Ahmad, Samantha Zorns, Abigail Straus, Sarah Sierra and Julian Paul Keenan * The Cognitive Neuroimaging Laboratory, Montclair State University, 207 Science Hall, Montclair, NJ 07043, USA; [email protected] (A.J.); [email protected] (B.B.); [email protected] (M.P.); [email protected] (V.M.); [email protected] (K.C.); [email protected] (J.B.); [email protected] (T.S.); [email protected] (V.M.); [email protected] (K.P.); [email protected] (N.A.); [email protected] (S.Z.); [email protected] (A.S.); [email protected] (S.S.) * Correspondence: [email protected] Abstract: While the desire to uncover the neural correlates of consciousness has taken numerous directions, self-face recognition has been a constant in attempts to isolate aspects of self-awareness. The neuroimaging revolution of the 1990s brought about systematic attempts to isolate the underlying neural basis of self-face recognition. These studies, including some of the first fMRI (functional magnetic resonance imaging) examinations, revealed a right-hemisphere bias for self-face recognition in a diverse set of regions including the insula, the dorsal frontal lobe, the temporal parietal junction, and the medial temporal cortex. In this systematic review, we provide confirmation of these data (which are correlational) which were provided by TMS (transcranial magnetic stimulation) and Citation: Janowska, A.; Balugas, B.; patients in which direct inhibition or ablation of right-hemisphere regions leads to a disruption or Pardillo, M.; Mistretta, V.; Chavarria, absence of self-face recognition. -
Illusory Own Body Perceptions: Case Reports and Relevance for Bodily Self-Consciousness Q
View metadata, citation and similar papers at core.ac.uk brought to you by CORE provided by Infoscience - École polytechnique fédérale de Lausanne Consciousness and Cognition 19 (2010) 702–710 Contents lists available at ScienceDirect Consciousness and Cognition journal homepage: www.elsevier.com/locate/concog Illusory own body perceptions: Case reports and relevance for bodily self-consciousness q Lukas Heydrich a,b, Sebastian Dieguez a, Thomas Grunwald c, Margitta Seeck b, Olaf Blanke a,b,* a Laboratory of Cognitive Neuroscience, Brain Mind Institute, Ecole Polytechnique Fédérale de Lausanne (EPFL), Switzerland b Department of Neurology, University Hospital Geneva, Switzerland c Swiss Epilepsy Center, Zurich, Switzerland article info abstract Keywords: Neurological disorders of body representation have for a long time suggested the impor- Epilepsy tance of multisensory processing of bodily signals for self-consciousness. One such group Self of disorders – illusory own body perceptions affecting the entire body – has been proposed Body representation to be especially relevant in this respect, based on neurological data as well as philosophical Depersonalization considerations. This has recently been tested experimentally in healthy subjects showing Multisensory Parietal cortex that integration of multisensory bodily signals from the entire body with respect to the Medial prefrontal cortex three aspects: self-location, first-person perspective, and self-location, is crucial for bodily self-consciousness. Here we present clinical and neuroanatomical data of two neurological patients with paroxysmal disorders of full body representation in whom only one of these aspects, self-identification, was abnormal. We distinguish such disorders of global body representation from related but distinct disorders and discuss their relevance for the neu- robiology of bodily self-consciousness. -
Neural Basis of Self-Initiative in Relation to Apathy in A
View metadata, citation and similar papers at core.ac.uk brought to you by CORE provided by University of Groningen University of Groningen Neural basis of self-initiative in relation to apathy in a student sample Kos, Claire; Klaasen, Nicky G.; Marsman, Jan-Bernard C.; Opmeer, Esther M.; Knegtering, Henderikus; Aleman, Andre; van Tol, Marie-Jose Published in: Scientific Reports DOI: 10.1038/s41598-017-03564-5 IMPORTANT NOTE: You are advised to consult the publisher's version (publisher's PDF) if you wish to cite from it. Please check the document version below. Document Version Publisher's PDF, also known as Version of record Publication date: 2017 Link to publication in University of Groningen/UMCG research database Citation for published version (APA): Kos, C., Klaasen, N. G., Marsman, J-B. C., Opmeer, E. M., Knegtering, H., Aleman, A., & van Tol, M-J. (2017). Neural basis of self-initiative in relation to apathy in a student sample. Scientific Reports, 7, [3264]. https://doi.org/10.1038/s41598-017-03564-5 Copyright Other than for strictly personal use, it is not permitted to download or to forward/distribute the text or part of it without the consent of the author(s) and/or copyright holder(s), unless the work is under an open content license (like Creative Commons). Take-down policy If you believe that this document breaches copyright please contact us providing details, and we will remove access to the work immediately and investigate your claim. Downloaded from the University of Groningen/UMCG research database (Pure): http://www.rug.nl/research/portal. -
Neuroscience of Self and Self-Regulation
PS62CH14-Heatherton ARI 22 November 2010 9:19 Neuroscience of Self and Self-Regulation Todd F. Heatherton Department of Psychological and Brain Sciences, Dartmouth College, Hanover, New Hampshire 03766; email: [email protected] Annu. Rev. Psychol. 2011. 62:363–90 Key Words The Annual Review of Psychology is online at self-awareness, theory of mind, need to belong, social neuroscience, psych.annualreviews.org neuroimaging, addiction This article’s doi: 10.1146/annurev.psych.121208.131616 Abstract by Dartmouth College on 12/08/10. For personal use only. Copyright c 2011 by Annual Reviews. As a social species, humans have a fundamental need to belong that en- All rights reserved courages behaviors consistent with being a good group member. Being 0066-4308/11/0110-0363$20.00 a good group member requires the capacity for self-regulation, which Annu. Rev. Psychol. 2011.62:363-390. Downloaded from www.annualreviews.org allows people to alter or inhibit behaviors that would place them at risk for group exclusion. Self-regulation requires four psychological com- ponents. First, people need to be aware of their behavior so as to gauge it against societal norms. Second, people need to understand how others are reacting to their behavior so as to predict how others will respond to them. This necessitates a third mechanism, which detects threat, es- pecially in complex social situations. Finally, there needs to be a mech- anism for resolving discrepancies between self-knowledge and social expectations or norms, thereby motivating behavior to resolve any con- flict that exists. This article reviews recent social neuroscience research on the psychological components that support the human capacity for self-regulation. -
Neural Basis of Self-Initiative in Relation to Apathy in a Student Sample
University of Groningen Neural basis of self-initiative in relation to apathy in a student sample Kos, Claire; Klaasen, Nicky G.; Marsman, Jan-Bernard C.; Opmeer, Esther M.; Knegtering, Henderikus; Aleman, Andre; van Tol, Marie-Jose Published in: Scientific Reports DOI: 10.1038/s41598-017-03564-5 IMPORTANT NOTE: You are advised to consult the publisher's version (publisher's PDF) if you wish to cite from it. Please check the document version below. Document Version Publisher's PDF, also known as Version of record Publication date: 2017 Link to publication in University of Groningen/UMCG research database Citation for published version (APA): Kos, C., Klaasen, N. G., Marsman, J-B. C., Opmeer, E. M., Knegtering, H., Aleman, A., & van Tol, M-J. (2017). Neural basis of self-initiative in relation to apathy in a student sample. Scientific Reports, 7, [3264]. https://doi.org/10.1038/s41598-017-03564-5 Copyright Other than for strictly personal use, it is not permitted to download or to forward/distribute the text or part of it without the consent of the author(s) and/or copyright holder(s), unless the work is under an open content license (like Creative Commons). The publication may also be distributed here under the terms of Article 25fa of the Dutch Copyright Act, indicated by the “Taverne” license. More information can be found on the University of Groningen website: https://www.rug.nl/library/open-access/self-archiving-pure/taverne- amendment. Take-down policy If you believe that this document breaches copyright please contact us providing details, and we will remove access to the work immediately and investigate your claim. -
''I Know You Are but What Am I?!'': Neural Bases of Self- and Social Knowledge Retrieval in Children and Adults
‘‘I Know You Are But What Am I?!’’: Neural Bases of Self- and Social Knowledge Retrieval in Children and Adults Jennifer H. Pfeifer, Matthew D. Lieberman, and Mirella Dapretto Abstract & Previous neuroimaging research with adults suggests that the more active during social than self-knowledge retrieval. Direct medial prefrontal cortex (MPFC) and the medial posterior parietal comparisons between children and adults indicated that children cortex (MPPC) are engaged during self-knowledge retrieval pro- activated the MPFC during self-knowledge retrieval to a much cesses. However, this has yet to be assessed in a developmental greater extent than adults. The particular regions of the MPPC sample. Twelve children and 12 adults (average age = 10.2 and involved varied between the two groups, with the posterior 26.1 years, respectively) reported whether short phrases de- precuneus engaged by adults, but the anterior precuneus and scribed themselves or a highly familiar other (Harry Potter) while posterior cingulate engaged by children. Only children activated undergoing functional magnetic resonance imaging. In both the MPFC significantly above baseline during self-knowledge re- children and adults, the MPFC was relatively more active during trieval. Implications for social cognitive development and the pro- self- than social knowledge retrieval, and the MPPC was relatively cessing functions performed by the MPFC are discussed. & INTRODUCTION larly think about—parallels one side of an ongoing The capacity to appreciate oneself as a distinct, unified, neuropsychological debate about whether the self is and enduring entity with abstract qualities such as ‘‘special’’ (Gillihan & Farah, 2005; Lieberman & Pfeifer, intelligence and agreeableness is one of the most unique 2005; Ochsner et al., 2005). -
The Common Neural Basis of Exerting Self-Control in Multiple Domains
The Common Neural Basis of Exerting Self-Control in Multiple Domains Jessica R. Cohen & Matthew D. Lieberman Department of Psychology, UCLA, Los Angeles, CA 90095, USA Introduction Self control can be defined as “the overriding or inhibiting of automatic, habitual, or innate behaviors, urges, emotions, or desires that would otherwise interfere with goal directed behavior” (Muraven et al., 2006). Without self-control, capricious and enjoyable decisions would be made, statements uttered, and actions taken. For example, if one has a deadline at work for an unpleasant project, he or she may have the inclination to leave work and do something fun instead of taking the responsible, yet dull, path of meeting the deadline. Or, if one has a strong urge to disclose to one’s boss his or her opinion of that horrendous project, the person may instead remain silent and sensibly agree to work late in order to competently finish the task at hand. While it is sometimes more desirable to follow one’s own whims, those actions could occur at the expense of practical and boring yet sensible decisions. Clearly it is important that some sort of internal control system be implemented in order to inhibit such impulses so that more appropriate decisions can be made and actions taken. When that control system is impaired problems occur, such as impulsive behavior in ADHD, gambling, poor financial decisions, substance abuse, etc. The negative behavioral and clinical manifestations of a lack of self-control underscore the importance of thoroughly understanding the basis of an intact control mechanism at many different levels of analysis, including cognitive, clinical, social, and neural. -
Neural Basis of Self and Other Representation in Autism: an Fmri Study of Self-Face Recognition
Neural Basis of Self and Other Representation in Autism: An fMRI Study of Self-Face Recognition Lucina Q. Uddin1,2*, Mari S. Davies2, Ashley A. Scott3, Eran Zaidel2, Susan Y. Bookheimer3, Marco Iacoboni3, Mirella Dapretto3 1 Department of Psychiatry, Stanford University School of Medicine, Stanford, California, United States of America, 2 Department of Psychology, University of California Los Angeles, Los Angeles, California, United States of America, 3 Ahmanson-Lovelace Brain Mapping Center, Semel Institute for Neuroscience and Human Behavior, Department of Psychiatry and Biobehavioral Sciences, David Geffen School of Medicine, University of California Los Angeles, Los Angeles, California, United States of America Abstract Background: Autism is a developmental disorder characterized by decreased interest and engagement in social interactions and by enhanced self-focus. While previous theoretical approaches to understanding autism have emphasized social impairments and altered interpersonal interactions, there is a recent shift towards understanding the nature of the representation of the self in individuals with autism spectrum disorders (ASD). Still, the neural mechanisms subserving self- representations in ASD are relatively unexplored. Methodology/Principal Findings: We used event-related fMRI to investigate brain responsiveness to images of the subjects’ own face and to faces of others. Children with ASD and typically developing (TD) children viewed randomly presented digital morphs between their own face and a gender-matched other face, and made ‘‘self/other’’ judgments. Both groups of children activated a right premotor/prefrontal system when identifying images containing a greater percentage of the self face. However, while TD children showed activation of this system during both self- and other- processing, children with ASD only recruited this system while viewing images containing mostly their own face. -
Medial Prefrontal Cortex Subserves Diverse Forms of Self-Reflection
SOCIAL NEUROSCIENCE, 2011, 6 (3), 211–218 PSNS Medial prefrontal cortex subserves diverse forms of self-reflection Neural Basis of Self-Reflection Adrianna C. Jenkins and Jason P. Mitchell Harvard University, Cambridge, MA, USA The ability to think about oneself—to self-reflect—is one of the defining features of the human mind. Recent research has suggested that this ability may be subserved by a particular brain region: the medial prefrontal cortex (MPFC). However, although humans can contemplate a variety of different aspects of themselves, including their stable personality traits, current feelings, and physical attributes, no research has directly examined the extent to which these different forms of self-reflection are subserved by common mechanisms. To address this question, participants were scanned using functional magnetic resonance imaging (fMRI) while making judgments about their own personality traits, current mental states, and physical attributes as well as those of another person. Whereas some brain regions responded preferentially during only one form of self-reflection, a robust region of MPFC was engaged preferentially during self-reflection across all three types of judgment. These results suggest that—although dissociable—diverse forms of self-referential thought draw on a shared cognitive process subserved by MPFC. INTRODUCTION Over the past decade, a particular brain region has repeatedly been linked to the distinction Throughout our lives, each of us effortlessly distin- between thinking about the self and thinking about guishes our own “self” from those around us. others. Specifically, the medial prefrontal cortex Although we may fumble the identities of other (MPFC) is consistently recruited by tasks that people (“Was it Bill or Jim whose daughter took those require participants to introspect about their own singing lessons?”), we rarely mistake ourselves for personality traits (e.g., “how adventurous am I?”) someone else. -
Brain Activity and Functional Coupling Changes Associated with Self-Reference Effect During Both Encoding and Retrieval
Brain Activity and Functional Coupling Changes Associated with Self-Reference Effect during Both Encoding and Retrieval. Nastassja Morel, Nicolas Villain, Géraldine Rauchs, Malo Gaubert, Pascale Piolino, Brigitte Landeau, Florence Mézenge, Béatrice Desgranges, Francis Eustache, Gaël Chételat To cite this version: Nastassja Morel, Nicolas Villain, Géraldine Rauchs, Malo Gaubert, Pascale Piolino, et al.. Brain Activity and Functional Coupling Changes Associated with Self-Reference Effect during Both Encod- ing and Retrieval.. PLoS ONE, Public Library of Science, 2014, 9 (3), pp.e90488. 10.1371/jour- nal.pone.0090488. inserm-00966855 HAL Id: inserm-00966855 https://www.hal.inserm.fr/inserm-00966855 Submitted on 27 Mar 2014 HAL is a multi-disciplinary open access L’archive ouverte pluridisciplinaire HAL, est archive for the deposit and dissemination of sci- destinée au dépôt et à la diffusion de documents entific research documents, whether they are pub- scientifiques de niveau recherche, publiés ou non, lished or not. The documents may come from émanant des établissements d’enseignement et de teaching and research institutions in France or recherche français ou étrangers, des laboratoires abroad, or from public or private research centers. publics ou privés. Brain Activity and Functional Coupling Changes Associated with Self-Reference Effect during Both Encoding and Retrieval Nastassja Morel1,2,3,4, Nicolas Villain1,2,3,4,Ge´raldine Rauchs1,2,3,4, Malo Gaubert1,2,3,4, Pascale Piolino5,6, Brigitte Landeau1,2,3,4, Florence Me´zenge1,2,3,4,Be´atrice Desgranges1,2,3,4, Francis Eustache1,2,3,4, Gae¨lChe´telat1,2,3,4* 1 Inserm, U1077, Caen, France, 2 Universite´ de Caen Basse-Normandie, UMR-S1077, Caen, France, 3 Ecole Pratique des Hautes Etudes, UMR-S1077, Caen, France, 4 CHU de Caen, U1077, Caen, France, 5 Universite´ de Paris Descartes, Laboratoire Me´moire et Cognition, Institut de Psychologie, Paris, France, 6 Inserm, U 894, Centre de Psychiatrie et Neurosciences, Paris, France Abstract Information that is processed with reference to oneself, i.e. -
Medial Prefrontal Cortex Subserves Diverse Forms of Self-Reflection
Running head: Neural basis of self-reflection Medial prefrontal cortex subserves diverse forms of self-reflection Adrianna C. Jenkins & Jason P. Mitchell Department of Psychology, Harvard University Keywords: self, medial prefrontal cortex, fMRI Address correspondence to: Adrianna Jenkins Department of Psychology Harvard University William James Hall 33 Kirkland Street Cambridge, MA 02138 Email: [email protected] Phone: 603.252.0541 Neural basis of self-reflection 2 ABSTRACT The ability to think about oneself!to self-reflect!is one of the defining features of the human mind. Recent research has suggested that this ability may be subserved by a particular brain region: the medial prefrontal cortex (MPFC). However, although humans can contemplate a variety of different aspects of themselves, including their stable personality traits, current feelings, and physical attributes, no research has directly examined the extent to which these different forms of self-reflection are subserved by common mechanisms. To address this question, participants were scanned using fMRI while making judgments about their own personality traits, current mental states, and physical attributes as well as those of another person. Whereas some brain regions responded preferentially during only one form of self-reflection, a robust region of MPFC was engaged preferentially during self-reflection across all three types of judgment. These results suggest that!although dissociable!diverse forms of self-referential thought draw on a shared cognitive process subserved by MPFC. Neural basis of self-reflection 3 INTRODUCTION Throughout our lives, each of us effortlessly distinguishes our own ‘self’ from those around us. Although we may fumble the identities of other people (“Was it Bill or Jim whose daughter took those singing lessons?”), we rarely mistake ourselves for someone else. -
A Translational Approach to the Neurobiology of Persecutory Ideation in Schizophrenia a DISSERTATION SUBMITTED to the FACULTY OF
A Translational Approach to the Neurobiology of Persecutory Ideation in Schizophrenia A DISSERTATION SUBMITTED TO THE FACULTY OF THE GRADUATE SCHOOL OF THE UNIVERSITY OF MINNESOTA BY Melissa Kay Johnson IN PARTIAL FULFILLMENT OF THE REQUIREMENTS FOR THE DEGREE OF DOCTOR OF PHILOSOPHY Advisor: Angus W. MacDonald III, Ph.D August 2011 © Melissa Kay Johnson, August/2011 Acknowledgements In these acknowledgements I thank my advisor, Angus MacDonald, for his mentorship. He has had a tremendous positive influence in my life. He is the most important teacher I ever had. I thank my husband who has supported me financially and emotionally over the past 10 years on this academic rollercoaster. We have grown so much together. I thank Dori Henderson, Madelyn Steen and Edward Patzelt for all of their help and for being such awesome people. I thank Kristen Haut and Rachel Force for their support and friendship. Finally, I thank the NARSAD foundation and Loiuse Francouer for their generous financial support of this project. i Dedication First, I dedicate this manuscript to my mother who would have been so insanely proud of me. Second, I dedicate this manuscript to my son. I’ve had so many people comment on how hard it must have been to have a child in graduate school. I honestly don’t know what I would have done without him. He gives me purpose and keeps me centered. Having him is the most important thing I have ever done. ii Table of Contents Acknowledgements……………………………………………………..……………....…i. Dedication……………………………………………………………………..………......ii Table of Contents………………………………………………………………...............iii List of Tables.....................................................................................................................vii List of Figures...................................................................................................................viii 1.