Possible Transport Evidence for Three-Dimensional Topological
Total Page:16
File Type:pdf, Size:1020Kb
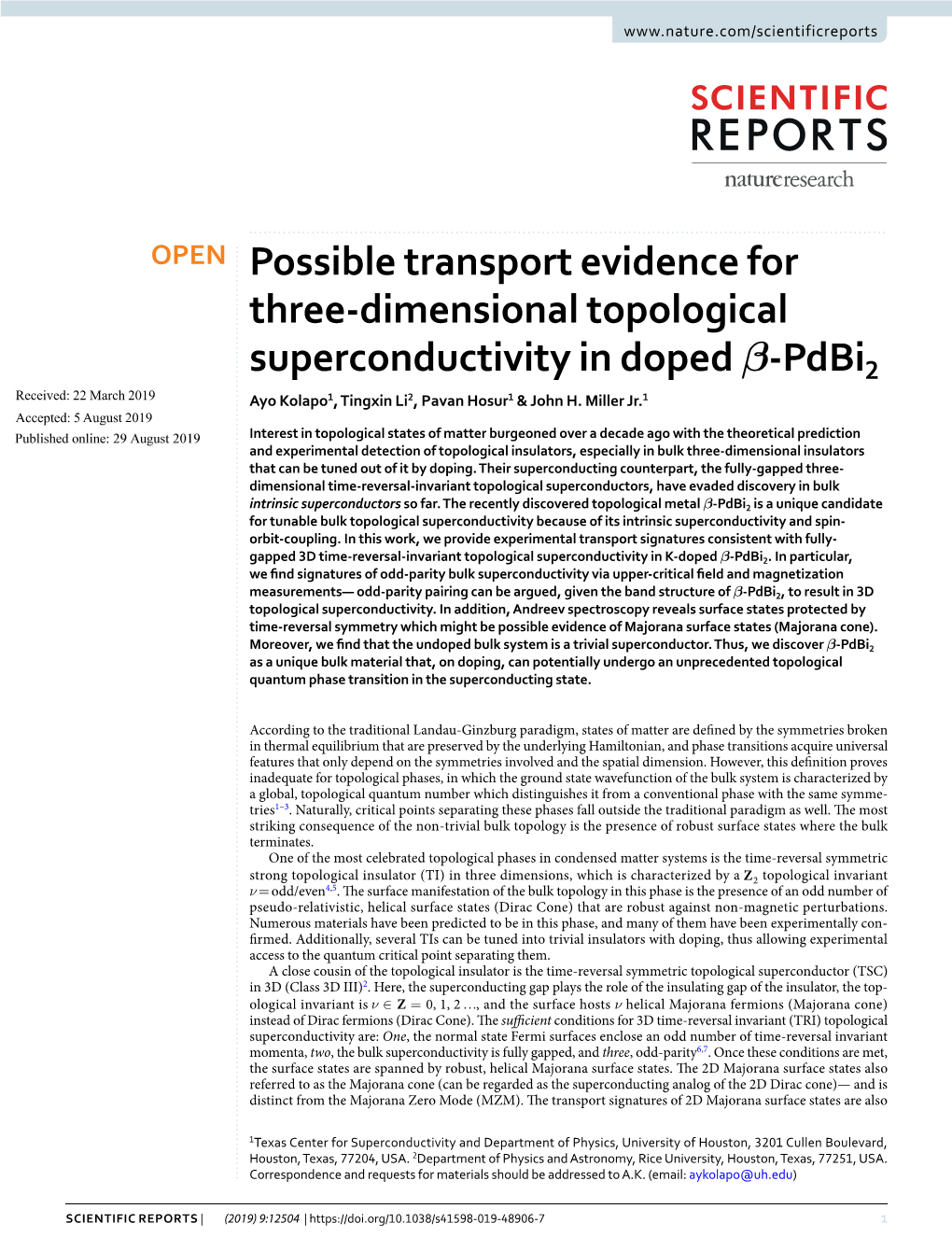
Load more
Recommended publications
-
Phase Coherence and Andreev Reflection in Topological Insulator Devices
PHYSICAL REVIEW X 4, 041022 (2014) Phase Coherence and Andreev Reflection in Topological Insulator Devices A. D. K. Finck,1 C. Kurter,1 Y. S. Hor, 2 and D. J. Van Harlingen1 1Department of Physics and Materials Research Laboratory, University of Illinois at Urbana-Champaign, Urbana, Illinois 61801, USA 2Department of Physics, Missouri University of Science and Technology, Rolla, Missouri 65409, USA (Received 3 June 2014; published 4 November 2014) Topological insulators (TIs) have attracted immense interest because they host helical surface states. Protected by time-reversal symmetry, they are robust to nonmagnetic disorder. When superconductivity is induced in these helical states, they are predicted to emulate p-wave pairing symmetry, with Majorana states bound to vortices. Majorana bound states possess non-Abelian exchange statistics that can be probed through interferometry. Here, we take a significant step towards Majorana interferometry by observing pronounced Fabry-Pérot oscillations in a TI sandwiched between a superconducting and a normal lead. For energies below the superconducting gap, we observe a doubling in the frequency of the oscillations, arising from an additional phase from Andreev reflection. When a magnetic field is applied perpendicular to the TI surface, a number of very sharp and gate-tunable conductance peaks appear at or near zero energy, which has consequences for interpreting spectroscopic probes of Majorana fermions. Our results demonstrate that TIs are a promising platform for exploring phase-coherent transport in a solid-state system. DOI: 10.1103/PhysRevX.4.041022 Subject Areas: Condensed Matter Physics, Superconductivity, Topological Insulators I. INTRODUCTION bound states (MBSs) can be realized in a topological insulator (TI) with induced superconductivity [6–8]. -
Circuit Theory of Crossed Andreev Reflection Physical Review B 74, 214510 ͑2006͒
PHYSICAL REVIEW B 74, 214510 ͑2006͒ Circuit theory of crossed Andreev reflection Jan Petter Morten,1,* Arne Brataas,1,2 and Wolfgang Belzig3 1Department of Physics, Norwegian University of Science and Technology, N-7491 Trondheim, Norway 2Centre for Advanced Study, Drammensveien 78, Oslo, N-0271 Norway 3Department of Physics, University of Konstanz, D-78457 Konstanz, Germany ͑Received 21 June 2006; revised manuscript received 11 September 2006; published 18 December 2006͒ We consider transport in a three-terminal device attached to one superconducting and two normal-metal terminals, using the circuit theory of mesoscopic superconductivity. We compute the nonlocal conductance of the current out of the first normal-metal terminal in response to a bias voltage between the second normal-metal terminal and the superconducting terminal. The nonlocal conductance is given by competing contributions from crossed Andreev reflection and electron cotunneling, and we determine the contribution from each process. The nonlocal conductance vanishes when there is no resistance between the superconducting terminal and the device, in agreement with previous theoretical work. Electron cotunneling dominates when there is a finite resistance between the device and the superconducting reservoir. Dephasing is taken into account, and the characteristic time scale is the particle dwell time. This gives rise to an effective Thouless energy. Both the conductance due to crossed Andreev reflection and electron cotunneling depend strongly on the Thouless energy. We suggest experimental determination of the conductance due to crossed Andreev reflection and electron cotunneling in measurement of both energy and charge flow into one normal-metal terminal in response to a bias voltage between the other normal-metal terminal and the superconductor. -
Spin-Polarized Supercurrents for Spintronics: a Review of Current Progressa
REVIEW ARTICLE Spin-polarized supercurrents for spintronics: a review of current progressa Matthias Eschrig Department of Physics, Royal Holloway, University of London, Egham Hill, Egham, Surrey TW20 0EX, United Kingdom E-mail: [email protected] Abstract. During the past 15 years a new field has emerged, which combines superconductivity and spintronics, with the goal to pave a way for new types of devices for applications combining the virtues of both by offering the possibility of long-range spin-polarized supercurrents. Such supercurrents constitute a fruitful basis for the study of fundamental physics as they combine macroscopic quantum coherence with microscopic exchange interactions, spin selectivity, and spin transport. This report follows recent developments in the controlled creation of long-range equal-spin triplet supercurrents in ferromagnets and its contribution to spintronics. The mutual proximity-induced modification of order in superconductor-ferromagnet hybrid structures introduces in a natural way such evasive phenomena as triplet superconductivity, odd-frequency pairing, Fulde-Ferrell-Larkin-Ovchinnikov pairing, long-range equal-spin supercurrents, π-Josephson junctions, as well as long-range magnetic proximity effects. All these effects were rather exotic before 2000, when improvements in nanofabrication and materials control allowed for a new quality of hybrid structures. Guided by pioneering theoretical studies, experimental progress evolved rapidly, and since 2010 triplet supercurrents are routinely produced and observed. We have entered a new stage of studying new phases of matter previously out of our reach, and of merging the hitherto disparate fields of superconductivity and spintronics to a new research direction: super-spintronics. a This is an author-created, un-copyedited version of an article accepted for publication in Reports on Progress in Physics. -
Andreev Reflection Enhancement In
CONTENTS - Continued PHYSICAL REVIEW B THIRD SERIES, VOLUME 97, NUMBER 5 FEBRUARY 2018-1(I) Andreev reflection enhancement in semiconductor-superconductor structures (10 pages) ...................... 054512 Shlomi Bouscher, Roni Winik, and Alex Hayat Proximity effect in normal-metal quasiparticle traps (18 pages) ............................................ 054513 A. Hosseinkhani and G. Catelani , Magnetic disorder and gap symmetry in the optimally electron-doped Sr(Fe Co)2As2 superconductor (11 pages) ........................................................................... 054514 Luminita Harnagea, Giri Mani, Rohit Kumar, and Surjeet Singh Heat capacity evidence for conventional superconductivity in the type-II Dirac semimetal PdTe2 (5 pages) ...... 054515 Amit and Yogesh Singh Effect of rotation on the elastic moduli of solid 4He (14 pages) ............................................ 054516 T. Tsuiki, D. Takahashi, S. Murakawa, Y. Okuda, K. Kono, and K. Shirahama Search for superfluidity in supercooled liquid parahydrogen (6 pages) ...................................... 054517 Massimo Boninsegni Tunable superconducting critical temperature in ballistic hybrid structures with strong spin-orbit coupling (14 pages) ................................................................................. 054518 Haakon T. Simensen and Jacob Linder Detecting sign-changing superconducting gap in LiFeAs using quasiparticle interference (10 pages) ............ 054519 D. Altenfeld, P. J. Hirschfeld, I. I. Mazin, and I. Eremin Field-induced coexistence -
Measurement of Spin Polarization by Andreev Reflection In
Measurement of Spin Polarization by Andreev Reflection in Ferromagnetic In1-xMnxSb Epilayers R. P. Panguluri, and B. Nadgornya Department of Physics and Astronomy, Wayne State University, Detroit, MI 48201 T. Wojtowicz, Department of Physics, University of Notre Dame, Notre Dame, IN 46556 and Institute of Physics, Polish Academy of Sciences, 02-668 Warsaw, Poland W.L. Lim, X. Liu, and J.K. Furdyna, Department of Physics, University of Notre Dame, Notre Dame, IN 46556 Abstract: We carried out Point Contact Andreev Reflection (PCAR) spin spectroscopy measurements on epitaxially-grown ferromagnetic In1-xMnxSb epilayers with a Curie temperature of ~9K. The spin sensitivity of PCAR in this material was demonstrated by parallel control studies on its non-magnetic analog, In1-yBeySb. We found the conductance curves of the Sn point contacts with In1-yBeySb to be fairly conventional, with the possible presence of proximity-induced superconductivity effects at the lowest temperatures. The experimental Z-values of interfacial scattering agreed well with the estimates based on the Fermi velocity mismatch between the semiconductor and the superconductor. These measurements provided control data for subsequent PCAR measurements on ferromagnetic In1-xMnxSb, which indicated spin polarization in In1- xMnxSb to be 52 ± 3%. a Electronic mail: [email protected] The recent emergence of III–Mn–V dilute ferromagnetic semiconductor alloys, 1 2 such as In1-xMnxAs and Ga1-xMnxAs , has already led to a number of exciting results relevant to spintronics applications. The ability to fabricate tunneling magneto-resistance (TMR) devices3 naturally integrated with the technologically important semiconductors, such as GaAs, makes these materials especially attractive. -
Andreev Reflection ( PCAR ) Spectroscopy Spin Polarization Superconducting Gap Pseudogaps ? Summary
Feb. 04, University of Virginia 1 2008 of IRON Superconductors Outline Introduction Point Contact (PC) spectroscopy Spin Transfer Torque ( STT ) Effects Point Contact Andreev Reflection ( PCAR ) spectroscopy Spin polarization Superconducting gap Pseudogaps ? Summary 4 Discovery of Superconductivity Liquefied Helium (1908), b.p. = 4.17 K Discovered superconductivity (1911) in Hg T C = 4.21 K Nobel prize (1913) Heike Kamerlingh Onnes ρ T 0 C T The saga of superconductivity began. 5 Perfect Conductivity and Perfect Diamagnetism Zero resistance Perfect conductivity I Persistent current I over 10 5 years Meissner effect Perfect diamagnetism No field inside SC 6 Type I and Type II Superconductors Type I (Pb, Al, etc) Type II (NbTi, Nb 3Sn, etc) B ≠ 0 B = 0 B = 0 Meissner (H < H C1 ) Meissner (H < H C) Mixed state (H C1 < H < H C2 ) HC2 (0) very large HC(0) ≈ 0.1 Tesla 7 Vortices in Mixed State Abrikosov Vortex Lattices in Superconductors (1957) Nobel Prize (2003) 2 ΦΦΦοοο = hc/2e ≈ 20 G-µm Hess (1989) (STM, NbSe ) Essmann (1967) (Bitter, Pb) 2 8 Josephson Effect 1962 Theoretical prediction of Josephson effect 1973 Nobel prize SC1 SC2 = φ Brian D. Josephson I(t) IC sin( (t)) φ: phase difference h − Φ = = .2 067833636 ×10 15 Wb 0 2e Superconducting Quantum Interference Device (SQUID ) Most sensitive detector 9 Bardeen-Cooper-Schrieffer (BCS) Theory (1957) e ξ e Cooper pairs Bardeen-Cooper-Schrieffer Nobel Prize, 1972 Superconducting Gap ∆∆∆(0)(0)(0) ∆∆∆ 2 /kBTC = 3.53 Eg = 2 10 Very large H c2 for Type II Superconductors High H C2 is necessary Superconducting magnets Magnetic levitation High TC is always beneficial 11 TC vs. -
Mesoscopic Superconductivitysuperconductivity Superconductivitysuperconductivity Inin Thethe Bulk:Bulk: Coopercooper Pairspairs
mesoscopicmesoscopic superconductivitysuperconductivity superconductivitysuperconductivity inin thethe bulk:bulk: CooperCooper pairspairs • electron-phonon interaction leads to an attraction of electrons • Cooper pairs: bound states of two electrons with opposite momentum and spin (size of Cooper pair: coherence length) • the net spin is zero and as a consequence they obey Bose- Einstein statistics: at low T all pairs condense in the lowest energy state (no Pauli exclusion !) • the superconducting state can then be described with a single, macroscopic wave function: Ψ = |Ψ| exp (iφ) |Ψ|2: density of Cooper pairs; φ: phase of the condensate • the pairing leads to an energy gap ∆ in the spectrum; the 2 2 1/2 density of states is Ns(E) = NN(E) E/(E -D ) • energy gap ∆ ≈ 1.75kBTC needed to excite a quasiparticle from the ground state (condensate) penetrationpenetration depthdepth andand criticalcritical magneticmagnetic fieldfield some numbers aluminum: Tc = 1.2 K; ∆ = 0.18 meV λL(0) = 50 nm 6 vF = 2 10 m/s ξ0 = 1.6 µm n = 1.8 1029 m3 superconductivitysuperconductivity inin particlesparticles <<<< λλ,,ξξ ?? spectroscopicspectroscopic measurementsmeasurements onon aa smallsmall superconductingsuperconducting inin particleparticle Determine the superconducting gap from the experiment? aa theoreticaltheoretical answeranswer eveneven--oddodd effecteffect Energy depends on the parity of the superconductors: 2 U = EC(Ne – αVG) + ∆i ∆i = 0 if N = 2n ∆i = ∆ if N = 2n+1 The ground state energy for odd n is ∆ above the minimum energy for even n. Even in an experiment on aluminum islands with 109 electrons, the parity of such a big number can be measured!! P. Lafarge et al. Phys. Rev. Lett. 70 (1993) 994 AndreevAndreev reflectionreflection atat aa NN--SS interfaceinterface • Clean N-S interface: E>∆ quasiparticle transport is possible; E<∆ single-particle tunneling is suppressed exponentially. -
Andreev Reflection in Two-Dimensional Relativistic Materials with Realistic
PHYSICAL REVIEW B 96, 054514 (2017) Andreev reflection in two-dimensional relativistic materials with realistic tunneling transparency in normal-metal/superconductor junctions Yung-Yeh Chang,1,* Chung-Yu Mou,2,3,4,† and Chung-Hou Chung1,3,4,‡ 1Department of Electrophysics, National Chiao Tung University, Hsinchu 30010, Taiwan, Republic of China 2Department of Physics, National Tsing Hua University, Hsinchu 30043, Taiwan, Republic of China 3Institute of Physics, Academia Sinica, Nankang 11529, Taiwan, Republic of China 4Physics Division, National Center for Theoretical Sciences, P. O. Box 2-131, Hsinchu 30043, Taiwan, Republic of China (Received 20 February 2017; published 17 August 2017) The Andreev conductance across realistic two-dimensional (2D) normal-metal (N)/superconductor (SC) junctions with a relativistic Dirac spectrum is theoretically investigated within the Blonder-Tinkham-Klapwijk formalism with tunable tunneling transparency. It is known that due to the effect of Klein tunneling, impurity potentials at the interface of 2D relativistic materials will enhance (not suppress) the tunneling and therefore are not suitable to model a realistic tunnel junction of these materials. Here, we propose a way to construct a more realistic tunnel junction by adding a narrow, homogeneous local strain, which effectively generates a δ-gauge potential and variations of electron hopping at the interface, to adjust the transparency of the N/SC junction. Remarkable suppression of the Andreev conductance is indeed observed in the graphene N/SC junction as the strength of the local strain increases. We also explore the Andreev conductance in a topological N/SC junction at the two inequivalent Dirac points and predict the distinctive behaviors for the conductance across the chiral-to-helical topological phase transition. -
Spin-Polarized Currents for Spintronic Devices: Point-Contact Andreev Reflection and Spin Filters
Sebastian von Oehsen (Autor) Spin-Polarized Currents for Spintronic Devices: Point-Contact Andreev Reflection and Spin Filters https://cuvillier.de/de/shop/publications/1624 Copyright: Cuvillier Verlag, Inhaberin Annette Jentzsch-Cuvillier, Nonnenstieg 8, 37075 Göttingen, Germany Telefon: +49 (0)551 54724-0, E-Mail: [email protected], Website: https://cuvillier.de 1. Introduction Semiconductor physics revolutionized the use of electronics and paved the way to complex new devices such as personal computers and mobile phones. The reduction in size created a giant increase of calculating power and consequently new areas of application. Today almost no part of our daily life goes without devices based on semiconductor physics. While the microcontrollers got smaller and smaller, another property of the electron than the charge came into the focus of attention: the spin. It is one of the puzzling foundations of quantum mechanics, that for a given direction in space there are only spin-up and/or spin-down electrons, nothing in between. It is the target of the emerging area of research, that was baptized spintronics by S.A. Wolf in 1996, to understand and utilize the electron’s spin to create useful sensors, memories, and logic devices with properties not possible with charge-based devices [Par04]. New devices may combine the standard microelectronics with spin-dependent effects that arise from the interaction between the spin of the carrier and a spin-dependent detector. Ferromagnets are naturally well suited as spin- dependent detectors, since in ferromagnets the degeneracy of the density of states of spin-up and spin-down electrons is lifted. -
Andreev-Klein Reflection in Graphene Ferromagnet-Superconductor Contacts
1960-11 ICTP Conference Graphene Week 2008 25 - 29 August 2008 Andreev-Klein reflection in graphene ferromagnet-superconductor contacts M. Zareyan Institute for Advanced Studies in Basic Sciences, Islamic Republic of Iran H. Mohammadpour Institute for Advanced Studies in Basic Sciences, Islamic Republic of Iran A.G. Moghaddam Institute for Advanced Studies in Basic Sciences, Islamic Republic of Iran Andreev-Klein reflection in graphene ferromagnet-superconductor contacts Malek Zareyan Hakimeh Mohammadpour and Ali G. Moghaddam ICTP Conference Graphene Week, August 2008 1 Andreev reflection in metallic NS junctions E Normal metal Superconductor ve s ke EF N(E) Cooper pair s kh vh [A. F. Andreev, Sov. JETP 19, 1228(1964)] 2 • Conversion of normal current into supercurrent: charge transfer by 2e Andreev Conductance: finite GNS of NS junction at voltages below the superconducting gap /e for an ideal interface at T=0 and V=0: GNS=2AR GNN Josephson current through SNS junctions: E E S N S Cooper pair transfer through N: Supercurrent through Andreev bound states: Critical (maximum) current + current-phase relation / 2 In a simple case / 2 I() Ic sin [G. E. Blonder, M. Tinkham, and T. M. Klapwijk, Phys. Rev. B 25, 4515 (1982); K. K. Likharev Rev. Mod. Phys. 52, 101 (1979);…. ] 3 •Momentum is changed by ~/vF << pF negligibly small for degenerate N << EF k k e h :AR is Retro Reflection Specular Normal Reflection Retro Andreev Reflection 4 Specular Andreev reflection in graphene NS junctions Superconducting correlation of Dirac electrons -
Andreev Reflection 36
Andreev Reflection Yasuhiro Asano Department of Applied Physics, Hokkaido University, Sapporo 060-8628, Japan. Email: [email protected] October, 2019 1 1 1Update version is available at http://zvine-ap.eng.hokudai.ac.jp/~asano/pdf1/lecture.pdf Contents 1 Introduction 4 1.1 Overview of superconducting phenomena . 4 2 Theoretical tools 10 2.1 Harmonic oscillator . 10 2.2 Free electrons in a metal . 14 2.3 Coherent state . 16 3 Mean-field theory of superconductivity 20 3.1 Pairing Hamiltonian . 20 3.2 Mean-field approximation . 22 3.3 Gap equation and thermodynamic properties . 24 3.4 Cooper pair . 27 3.5 Magnetic properties . 29 4 Andreev reflection 35 4.1 Conductance in an NS junction . 36 4.2 Retroreflectivity . 42 4.3 Josephson current in an SIS junction . 43 5 Unconventional superconductor 49 5.1 Conductance in an NS junction . 51 5.2 Surface Andreev bound states . 53 5.3 Josephson current in an SIS junction . 56 5.4 Andreev bound states in an SIS junction . 57 5.5 Topological classification . 59 6 Various Josephson junctions 64 6.1 Singlet-singlet junction . 65 6.2 Triplet-triplet junction . 68 6.3 Singlet-triplet junction . 70 6.4 Chiral superconductors . 73 6.5 Helical superconductors . 74 6.6 Noncentrosymmetric superconductor . 75 A Pair potential near the transition temperature 76 B Landauer formula for electric conductance 78 2 CONTENTS 3 C Mean-field theory in real space 83 D Reflection coefficients in one-dimensional junctions 86 Chapter 1 Introduction Superconductivity is a macroscopic quantum phenomenon discovered by H. -
Quantum Critical Scaling and Fluctuations in Kondo Lattice Materials
Supporting Information: Quantum critical scaling and fluctuations in Kondo lattice materials Yi-feng Yanga,b,c, David Pinesd, and Gilbert Lonzariche a b Beijing National Laboratory for Condensed Matter Physics and Institute of Physics, Chinese Academy of Sciences, Beijing 100190, China; Collaborative Innovation c d Center of Quantum Matter, Beijing 100190, China; School of Physical Sciences, University of Chinese Academy of Sciences, Beijing 100190, China; Santa Fe Institute, e Santa Fe, NM 87501, USA; Cavendish Laboratory, Department of Physics, Cambridge University, Cambridge CB3 0HE, UK 1. Probes of scaling and fluctuations We discuss here the experimental probes that have been used to identify the scaling behavior shown in the main text (1). ∗ The NMR Knight shift. While there are a number of candidate experimental signatures of T (2), and the scaling behavior of ∗ the Kondo liquid found below it (3), only one is unambiguous: the Knight shift experiments which show that above T the ∗ Knight shift follows the uniform spin susceptibility (and measures local moment behavior) and that it ceases to do this at T ∗ because a second component, the heavy electron liquid, emerges. As Curro et al. showed (4), below T one can disentangle the heavy electron component from the local moment contribution to the Knight shift and show that it displays universal scaling behavior as it varies logarithmically with temperature down to a temperature, TQC . Of all the materials that exhibit a Knight shift anomaly that enables one to follow heavy electron behavior directly, the variation of T ∗ with a control parameter (pressure, applied magnetic field, or doping), has been followed in detail in only one material, CeRhIn5, where pressure is the ∗ control parameter (5), while experiments on this and other materials show that T does not change appreciably on applying a magnetic field (6).