Ribonuclease Activity
Total Page:16
File Type:pdf, Size:1020Kb
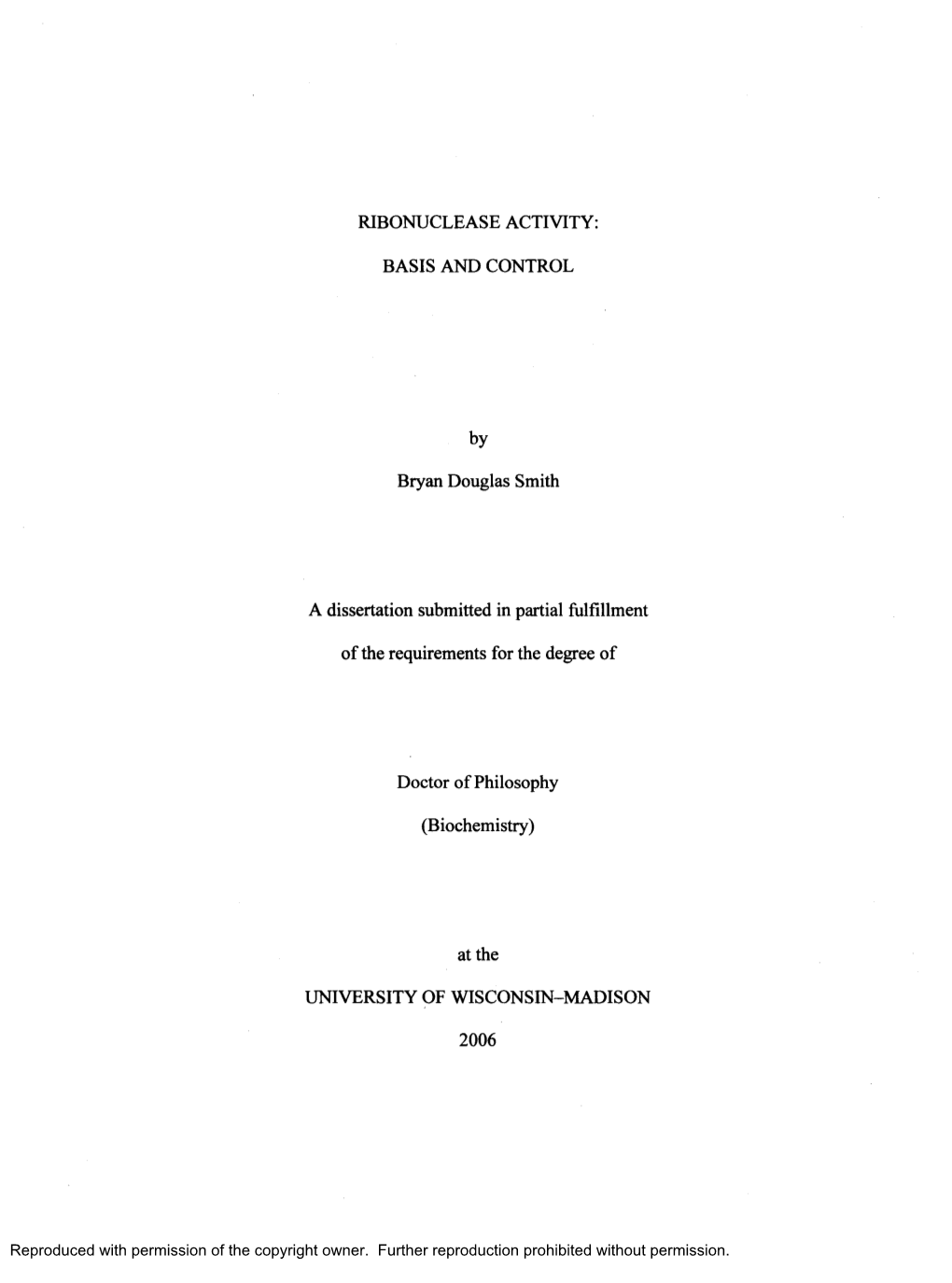
Load more
Recommended publications
-
(12) United States Patent (10) Patent No.: US 6,686,188 B2 Gu Et Al
US0066861.88B2 (12) United States Patent (10) Patent No.: US 6,686,188 B2 Gu et al. (45) Date of Patent: Feb. 3, 2004 (54) POLYNUCLEOTIDE ENCODING A HUMAN 4,469,863 A 9/1984 Tso et al. MYOSIN-LIKE POLYPEPTIDE EXPRESSED 4,476,301 A 10/1984 Imbach et al. PREDOMINANTLY IN HEART AND MUSCLE 4,708,871 A 11/1987 Geysen 5,023.243 A 6/1991 Tullis 5,034,506 A 7/1991 Summerton et al. (75) Inventors: Yizhong Gu, Sunnyvale, CA (US); 5,166,315 A 11/1992 Summerton et al. Yonggang Ji, San Mateo, CA (US); 5,177,196 A 1/1993 Meyer, Jr. et al. Sharron Gaynor Penn, San Mateo, CA 5,185,444 A 2/1993 Summerton et al. (US); David Kagen Hanzel, Palo Alto, 5,186,042 A 2/1993 Miyazaki CA (US); David Russell Rank, 5,188,897 A 2/1993 Suhadolnik et al. Fremont, CA (US); Wensheng Chen, 5,214,134 A 5/1993 Weis et al. Mountain View, CA (US); Mark E. 5,216,141 A 6/1993 Benner Shannon, Livermore, CA (US) 5,235,033 A 8/1993 Summerton et al. 5,264,423 A 11/1993 Cohen et al. (73) Assignee: Amersham PLC, Buckinghamshire 5,264,562 A 11/1993 Matteucci 5,264,564 A 11/1993 Matteucci (GB) 5,272,071 A 12/1993 Chappel (*) Notice: Subject to any disclaimer, the term of this 5,276,019 A 1/1994 Cohen et al. patent is extended or adjusted under 35 5,278.302 A 1/1994 Caruthers et al. -
Molecular Genetic Approaches to Decrease Mis-Incorporation of Non
Molecular genetic approaches to decrease mis-incorporation of non-canonical branched chain amino acids into a recombinant protein in Escherichia coli Ángel Córcoles García Molecular genetic approaches to decrease mis- incorporation of non-canonical branched chain amino acids into a recombinant protein in Escherichia coli Ángel Córcoles García - Dissertation Abstract II Molecular genetic approaches to decrease mis-incorporation of non-canonical branched chain amino acids into a recombinant protein in Escherichia coli vorgelegt von M. Sc. Ángel Córcoles García ORCID: 0000-0001-9300-5780 von der Fakultät III-Prozesswissenschaften der Technischen Universität Berlin zur Erlangung des akademischen Grades Doktor der Naturwissenschaften - Dr. rer. nat. - genehmigte Dissertation Promotionsausschuss: Vorsitzender: Prof. Dr. Juri Rappsilber, Institut für Biotechnologie, TU Berlin, Berlin Gutachter: Prof. Dr. Peter Neubauer, Institut für Biotechnologie, TU Berlin, Berlin Gutachter: Prof. Dr. Pau Ferrer, Universitat Autònoma de Barcelona, Bellaterra (Cerdanyola del Vallès), Spain Gutachter: Dr. Heinrich Decker, Sanofi-Aventis Deutschland GmbH, Frankfurt am Main Tag der wissenschaftlichen Aussprache: 11. Dezember 2019 Berlin 2020 Molecular genetic approaches to decrease mis-incorporation of non-canonical branched chain amino acids into a recombinant protein in Escherichia coli Ángel Córcoles García Abstract The incorporation of non-canonical branched chain amino acids (ncBCAA) such as norleucine, norvaline and β-methylnorleucine into recombinant proteins during E.coli production processes has become a crucial matter of contention in the pharmaceutical industry, since such mis-incorporation can lead to production of altered proteins, having non optimal characteristics. Hence, a need exists for novel strategies valuable for preventing the mis-incorporation of ncBCAA into recombinant proteins. This work presents the development of novel E. -
Transmembrane Domain 1 of Human Organic Anion Transporting Polypeptide 2B1 Is
Molecular Pharmacology Fast Forward. Published on June 5, 2018 as DOI: 10.1124/mol.118.111914 This article has not been copyedited and formatted. The final version may differ from this version. MOL #111914 Transmembrane domain 1 of human organic anion transporting polypeptide 2B1 is essential for transporter function and stability Zihui Fang, Jiujiu Huang, Jie Chen, Shaopeng Xu, Zhaojian Xiang, Mei Hong College of Life Sciences, South China Agricultural University, Guangzhou, China (Z.F., J.H., Downloaded from J.C, S.X, Z.X, M.H.), and Guangdong Provincial Key Laboratory of Protein Function and Regulation in Agricultural Organisms, South China Agricultural University, Guangzhou, molpharm.aspetjournals.org China (J.H., M.H.) at ASPET Journals on September 29, 2021 1 Molecular Pharmacology Fast Forward. Published on June 5, 2018 as DOI: 10.1124/mol.118.111914 This article has not been copyedited and formatted. The final version may differ from this version. MOL #111914 Running title: Transmembrane domain 1 is important for OATP2B1 function Address correspondence to: Mei Hong, College of Life Sciences, South China Agricultural University, Guangzhou, China, Tel: (8620)8528-0901; Fax: (8620)8528-2180; Email: [email protected] Downloaded from Number of text pages: 30 molpharm.aspetjournals.org Number of tables: 1 Number of figures: 8 Number of references: 37 at ASPET Journals on September 29, 2021 Number of words in the Abstract: 237 Number of words in the Introduction: 682 Number of words in the Discussion: 1113 Abbreviations: BFA1: bafilomycin A1; ES: estrone-3-sulfate; NHS-SS-biotin: sulfosuccinimidyl 2-(biotinamido) -ethyl-1, 3-dithiopropionate; OATP: organic anion transporting polypeptide; TM: transmembrane domain 2 Molecular Pharmacology Fast Forward. -
Purification, Kinetic Characterization, and Site-Directed Mutagenesis of Methanothermobacter Thermautotrophicus RFAP Synthase Produced in Escherichia Coli
AIMS Microbiology, 5(3): 186–204. DOI: 10.3934/microbiol.2019.3.186 Received: 02 May 2019 Accepted: 15 July 2019 Published: 23 July 2019 http://www.aimspress.com/journal/microbiology Research article Purification, kinetic characterization, and site-directed mutagenesis of Methanothermobacter thermautotrophicus RFAP Synthase Produced in Escherichia coli Matthew E. Bechard1, Payam Farahani2, Dina Greene3, Anna Pham2, Andrew Orry4 and Madeline E. Rasche2,* 1 Department of Pathology, Microbiology and Immunology, Vanderbilt University Medical Center, Nashville, TN 37232 2 Chemistry and Biochemistry Department, California State University at Fullerton, 800 North State College Blvd., Fullerton, CA 92834 3 Northern California Regional Laboratories, The Permanente Medical Group, Berkeley, CA 94710 4 Molsoft L.L.C., 11199 Sorrento Valley Road, S209, San Diego, CA 92121 Correspondence: Email: [email protected]; Tel: 6572783885; Fax: 6572785613. Abstract: Methane-producing archaea are among a select group of microorganisms that utilize tetrahydromethanopterin (H4MPT) as a one-carbon carrier instead of tetrahydrofolate. In H4MPT biosynthesis, β-ribofuranosylaminobenzene 5’-phosphate (RFAP) synthase catalyzes the production of RFAP, CO2, and pyrophosphate from p-aminobenzoic acid (pABA) and phosphoribosyl-pyrophosphate (PRPP). In this work, to gain insight into amino acid residues required for substrate binding, RFAP synthase from Methanothermobacter thermautotrophicus was produced in Escherichia coli, and site-directed mutagenesis was used to alter arginine 26 (R26) and aspartic acid 19 (D19), located in a conserved sequence of amino acids resembling the pABA binding site of dihydropteroate synthase. Replacement of R26 with lysine increased the KM for pABA by an order of magnitude relative to wild-type enzyme without substantially altering the KM for PRPP. -
Genetics of Lipedema: New Perspectives on Genetic Research and Molecular Diagnoses S
European Review for Medical and Pharmacological Sciences 2019; 23: 5581-5594 Genetics of lipedema: new perspectives on genetic research and molecular diagnoses S. PAOLACCI1, V. PRECONE2, F. ACQUAVIVA3, P. CHIURAZZI4,5, E. FULCHERI6,7, M. PINELLI3,8, F. BUFFELLI9,10, S. MICHELINI11, K.L. HERBST12, V. UNFER13, M. BERTELLI2; GENEOB PROJECT 1MAGI’S LAB, Rovereto (TN), Italy 2MAGI EUREGIO, Bolzano, Italy 3Department of Translational Medicine, Section of Pediatrics, Federico II University, Naples, Italy 4Istituto di Medicina Genomica, Fondazione A. Gemelli, Università Cattolica del Sacro Cuore, Rome, Italy 5UOC Genetica Medica, Fondazione Policlinico Universitario “A. Gemelli” IRCCS, Rome, Italy 6Fetal and Perinatal Pathology Unit, IRCCS Istituto Giannina Gaslini, Genoa, Italy 7Department of Integrated Surgical and Diagnostic Sciences, University of Genoa, Genoa, Italy 8Telethon Institute of Genetics and Medicine (TIGEM), Pozzuoli, Italy 9Fetal and Perinatal Pathology Unit, IRCCS Istituto Giannina Gaslini, Genoa, Italy 10Department of Neuroscience, Rehabilitation, Ophthalmology, Genetics and Maternal-Infantile Sciences, University of Genoa, Genoa, Italy 11Department of Vascular Rehabilitation, San Giovanni Battista Hospital, Rome, Italy 12Department of Medicine, University of Arizona, Tucson, AZ, USA 13Department of Developmental and Social Psychology, Faculty of Medicine and Psychology, Sapienza University of Rome, Rome, Italy Abstract. – OBJECTIVE: The aim of this quali- Introduction tative review is to provide an update on the cur- rent understanding of the genetic determinants of lipedema and to develop a genetic test to dif- Lipedema is an underdiagnosed chronic debil- ferentiate lipedema from other diagnoses. itating disease characterized by bruising and pain MATERIALS AND METHODS: An electronic and excess of subcutaneous adipose tissue of the search was conducted in MEDLINE, PubMed, and legs and/or arms in women during or after times Scopus for articles published in English up to of hormone change, especially in puberty1. -
Fixation of Deleterious Mutations at Critical Positions in Human Proteins
Fixation of Deleterious Mutations at Critical Positions in Human Proteins Author Sankarasubramanian, Sankar Published 2011 Journal Title Molecular Biology and Evolution DOI https://doi.org/10.1093/molbev/msr097 Copyright Statement © 2011 Oxford University Press. This is a pre-copy-editing, author-produced PDF of an article accepted for publication in Molecular Biology and Evolution following peer review. The definitive publisher-authenticated version Fixation of Deleterious Mutations at Critical Positions inHuman Proteins, Molecular Biology and Evolution, (2011) 28 (9): 2687-2693 is available online at: http:// dx.doi.org/10.1093/molbev/msr097 Downloaded from http://hdl.handle.net/10072/44247 Griffith Research Online https://research-repository.griffith.edu.au Research article March 29, 2011 Fixation of deleterious mutations at critical positions in human proteins Sankar Subramanian Griffith School of Environment, Griffith University, 170 Kessels Road, Nathan Qld 4111, Australia Keywords: human evolution, natural selection, deleterious mutations, fixation, WGA and population genetics theory Running head: Fixation of deleterious mutations in human Title length: 73 characters (including spaces) Abstract length: 245 words Total length of text: 26,662 characters (including spaces) Total page requirement: 5.5 pages Number of figures: 3 Number of tables: 2 Number of references: 27 Address for correspondence: Dr. Sankar Subramanian Griffith School of Environment Griffith University 170 Kessels Road Nathan QLD 4111 Australia Phone: +61-7-3735 7495 Fax: +61-7-3735 7459 E-mail: [email protected] 1 Abstract Deleterious mutations associated with human diseases are predominantly found in conserved positions and positions that are essential for the structure and/or function of proteins. -
Small Molecule Diselenides As Probes of Oxidative Protein Folding
Research Collection Doctoral Thesis Small molecule diselenides as probes of oxidative protein folding Author(s): Beld, Joris Publication Date: 2009 Permanent Link: https://doi.org/10.3929/ethz-a-005950993 Rights / License: In Copyright - Non-Commercial Use Permitted This page was generated automatically upon download from the ETH Zurich Research Collection. For more information please consult the Terms of use. ETH Library Diss. ETH No. 18510 Small molecule diselenides as probes of oxidative protein folding. A dissertation submitted to ETH Zürich For the degree of Doctor of Sciences Presented by Joris Beld MSc. University of Twente, Enschede Born February 15, 1978 Citizen of the Netherlands Accepted on the recommendation of Prof. Dr. Donald Hilvert, examiner Prof. Dr. Bernhard Jaun, co‐examiner Zürich 2009 How to work better. Do one thing at a time Know the problem Learn to listen Learn to ask questions Distinguish sense from nonsense Accept change as inevitable Admit mistakes Say it simple Be calm Smile Peter Fischli and David Weiss, 1991 To my family. Publications Parts of this thesis have been published. Beld, J., Woycechowsky, K.J., and Hilvert, D. Small molecule diselenides catalyze oxidative protein folding in vivo, submitted (2009) Beld, J., Woycechowsky, K.J., and Hilvert, D. Diselenide resins for oxidative protein folding, patent application EP09013216 (2009) Beld, J., Woycechowsky, K. J., and Hilvert, D. Catalysis of oxidative protein folding by small‐ molecule diselenides, Biochemistry 47, 6985‐6987 (2008) Beld, J., Woycechowsky, K.J., and Hilvert, D. Selenocysteine as a probe of oxidative protein folding, Oxidative folding of Proteins and Peptides, edited by J. -
Sequence and Structural Similarities Between the Leucine-Specific Binding Protein and Leucyl-Trna Synthetase of Escherichia Coil (Evolution/Protein Structure) RUFUS M
Proc. Natl. Acad. Sci. USA Vol. 87, pp. 4561-4565, June 1990 Biochemistry Sequence and structural similarities between the leucine-specific binding protein and leucyl-tRNA synthetase of Escherichia coil (evolution/protein structure) RUFUS M. WILLIAMSON AND DALE L. OXENDER* Department of Biological Chemistry, University of Michigan Medical School, Ann Arbor, MI 48109-0606 Communicated by M. J. Coon, March 12, 1990 (received for review December 21, 1989) ABSTRACT A role for the leucyl-tRNA synthetase (EC larity to the carboxyl-terminal domain of the leucine-specific 6.1.1.4) has been established for regulating the transport of binding protein. leucine across the inner membrane of Escherchia coli by the leucine, isoleucine, valine (LIV-I) transport system. This trans- RESULTS AND DISCUSSION port system is mediated by interactions of periplasmic binding Sequence Similarity Between Leucyl-tRNA Synthetase and proteins with a complex of membrane-associated proteins, and Leucine-Specific Binding Protein. The leucine-specific bind- transcription of the high-affinity branched-chain amino acid ing protein is synthesized as a 369-amino acid precursor (14). transport system genes is repressed by growth ofE. coli on high The precursor undergoes processing to a mature form con- levels of leucine. We now report results from sequence compar- taining 346 amino acids by cleavage of a signal peptide at the isons and structural modeling studies, which indicate that the amino terminus (15). Leucyl-tRNA synthetase is a monomer leucine-specific binding protein, one of the periplasmic compo- of 860 amino acids (16). A region of the leucine-specific nents of the LIV-I transport system, contains a 121-residue binding protein, 121 residues in length and comprising 36% of stretch, representing 36% ofthe mature protein, which displays the mature binding protein, was found to display similarity both sequence and structural similarities to a region within the with two regions of leucyl-tRNA synthetase that are sepa- putative nucleotide-binding domain of leucyl-tRNA synthetase. -
In-Cell Synthesis of Bioorthogonal Alkene Tag S-Allyl-Homocysteine and Its Coupling with Reprogrammed Translation
International Journal of Molecular Sciences Article In-Cell Synthesis of Bioorthogonal Alkene Tag S-Allyl-Homocysteine and Its Coupling with Reprogrammed Translation Saba Nojoumi 1, Ying Ma 1, Sergej Schwagerus 2,3, Christian P. R. Hackenberger 2,3 and Nediljko Budisa 1,4,* 1 Institut für Chemie, Technische Universität Berlin, Müller-Breslau-Str. 10, D-10623 Berlin, Germany; [email protected] (S.N.); [email protected] (Y.M.) 2 Institut für Chemie der Humboldt-Universität zu Berlin, Brook-Taylor-Str. 2, D-12489 Berlin, Germany; [email protected] (S.S.); [email protected] (C.P.R.H.) 3 Leibniz-Forschungsinstitut für Molekulare Pharmakologie (FMP), Campus Berlin-Buch, Robert-Roessle-Str. 10, D-13125 Berlin, Germany 4 Chair of Chemical Synthetic Biology, Department of Chemistry, University of Manitoba, 144 Dysart Rd, Winnipeg, MB R3T 2N2, Canada * Correspondence: [email protected] or [email protected]; Tel.: +49-30-314-28821 or +1-204-474-9178 Received: 18 April 2019; Accepted: 7 May 2019; Published: 9 May 2019 Abstract: In this study, we report our initial results on in situ biosynthesis of S-allyl-l-homocysteine (Sahc) by simple metabolic conversion of allyl mercaptan in Escherichia coli, which served as the host organism endowed with a direct sulfhydration pathway. The intracellular synthesis we describe in this study is coupled with the direct incorporation of Sahc into proteins in response to methionine codons. Together with O-acetyl-homoserine, allyl mercaptan was added to the growth medium, followed by uptake and intracellular reaction to give Sahc. Our protocol efficiently combined the in vivo synthesis of Sahc via metabolic engineering with reprogrammed translation, without the need for a major change in the protein biosynthesis machinery. -
Transmembrane Domain 1 of Human Organic Anion Transporting Polypeptide 2B1 Is Essential for Transporter Function and Stability S
Supplemental material to this article can be found at: http://molpharm.aspetjournals.org/content/suppl/2018/06/05/mol.118.111914.DC1 1521-0111/94/2/842–849$35.00 https://doi.org/10.1124/mol.118.111914 MOLECULAR PHARMACOLOGY Mol Pharmacol 94:842–849, August 2018 Copyright ª 2018 by The American Society for Pharmacology and Experimental Therapeutics Transmembrane Domain 1 of Human Organic Anion Transporting Polypeptide 2B1 Is Essential for Transporter Function and Stability s Zihui Fang,1 Jiujiu Huang,1 Jie Chen, Shaopeng Xu, Zhaojian Xiang, and Mei Hong College of Life Sciences (Z.F., J.H., J.C., S.X., Z.X., M.H.) and Guangdong Provincial Key Laboratory of Protein Function and Regulation in Agricultural Organisms (J.H., M.H.), South China Agricultural University, Guangzhou, China Received January 29, 2018; accepted May 24, 2018 Downloaded from ABSTRACT Organic anion transporting polypeptides (OATPs, gene symbol OATP2B1 revealed that substitution of L58 with alanine SLCO) are important membrane transporter proteins that dramatically altered the Km value, and mutation of V52, H55, mediate the uptake of wide ranges of endogenous and Q59, and L69 resulted in significantly reduced substrate exogenous compounds. OATP2B1 has been found in multiple turnover number, whereas A61V, Q62A, and S66A exhibited molpharm.aspetjournals.org organs and tissues, including the liver, small intestine, kidney, significantchangeinbothKm and Vmax values. In addition, brain, placenta, heart, skin, as well as skeletal muscle, and is phenylalanine at position 51 seems to play an important role in proposed to be involved in the uptake of orally administered maintaining proper folding of OATP2B1 because alanine re- drugs. -
Cytogenetics
Scope The Atlas of Genetics and Cytogenetics in Oncology and Haematology is a peer reviewed on-line journal in open access, devoted to genes, cytogenetics, and clinical entities in cancer, and cancer-prone diseases. It presents structured review articles ("cards") on genes, leukaemias, solid tumours, cancer-prone diseases, more traditional review articles on these and also on surrounding topics ("deep insights"), case reports in hematology, and educational items in the various related topics for students in Medicine and in Sciences. Editorial correspondance Jean-Loup Huret Genetics, Department of Medical Information, University Hospital F-86021 Poitiers, France tel +33 5 49 44 45 46 or +33 5 49 45 47 67 [email protected] or [email protected] Staff Mohammad Ahmad, Mélanie Arsaban, Mikael Cordon, Isabelle Dabin, Marie-Christine Jacquemot-Perbal, Maureen Labarussias, Anne Malo, Catherine Morel-Pair, Laurent Rassinoux, Sylvie Yau Chun Wan - Senon, Alain Zasadzinski. Philippe Dessen is the Database Director, and Alain Bernheim the Chairman of the on-line version (Gustave Roussy Institute – Villejuif – France). The Atlas of Genetics and Cytogenetics in Oncology and Haematology (ISSN 1768-3262) is published 12 times a year by ARMGHM, a non profit organisation, and by the INstitute for Scientific and Technical Information of the French National Center for Scientific Research (INIST-CNRS) since 2008. The Atlas is hosted by INIST-CNRS (http://www.inist.fr) http://AtlasGeneticsOncology.org © ATLAS - ISSN 1768-3262 Atlas Genet Cytogenet Oncol Haematol. 2009; 13(5) Atlas of Genetics and Cytogenetics in Oncology and Haematology OPEN ACCESS JOURNAL AT INIST-CNRS Scope The Atlas of Genetics and Cytogenetics in Oncology and Haematology is a peer reviewed on-line journal in open access, devoted to genes, cytogenetics, and clinical entities in cancer, and cancer-prone diseases. -
Gs Protein Peptidomimetics As Allosteric Modulators of the Β2
RSC Advances View Article Online PAPER View Journal | View Issue Gs protein peptidomimetics as allosteric modulators of the b -adrenergic receptor† Cite this: RSC Adv.,2018,8, 2219 2 Lotte-Emilie Boyhus,a Mia Danielsen, a Nina Smidt Bengtson,a Micha Ben Achim Kunze,b Xavier Kubiak,c Tjerk J. Sminia,a Jacob Hartvig Løper,a Phuong Thu Tran,a Kresten Lindorff-Larsen, b Søren G. F. Rasmussen,c Jesper Mosolff Mathiesen‡*a and Daniel Sejer Pedersen ‡*a A series of Gs protein peptidomimetics were designed and synthesised based on the published X-ray crystal structure of the active state b2-adrenergic receptor (b2AR) in complex with the Gs protein (PDB 3SN6). We hypothesised that such peptidomimetics may function as allosteric modulators that target the intracellular Gs protein binding site of the b2AR. Peptidomimetics were designed to mimic the 15 residue C-terminal a- helix of the Gs protein and were pre-organised in a helical conformation by (i, i + 4)-stapling using copper catalysed azide alkyne cycloaddition. Linear and stapled peptidomimetics were analysed by circular dichroism (CD) and characterised in a membrane-based cAMP accumulation assay and in a bimane Creative Commons Attribution 3.0 Unported Licence. fluorescence assay on purified b2AR. Several peptidomimetics inhibited agonist isoproterenol (ISO) induced cAMP formation by lowering the ISO maximal efficacy up to 61%. Moreover, some peptidomimetics were found to significantly decrease the potency of ISO up to 39-fold. In the bimane Received 23rd October 2017 fluorescence assay none of the tested peptidomimetics could stabilise an active-like conformation of Accepted 4th December 2017 b2AR.