Revision 1 Raman Spectroscopic Quantification of Tetrahedral Boron
Total Page:16
File Type:pdf, Size:1020Kb
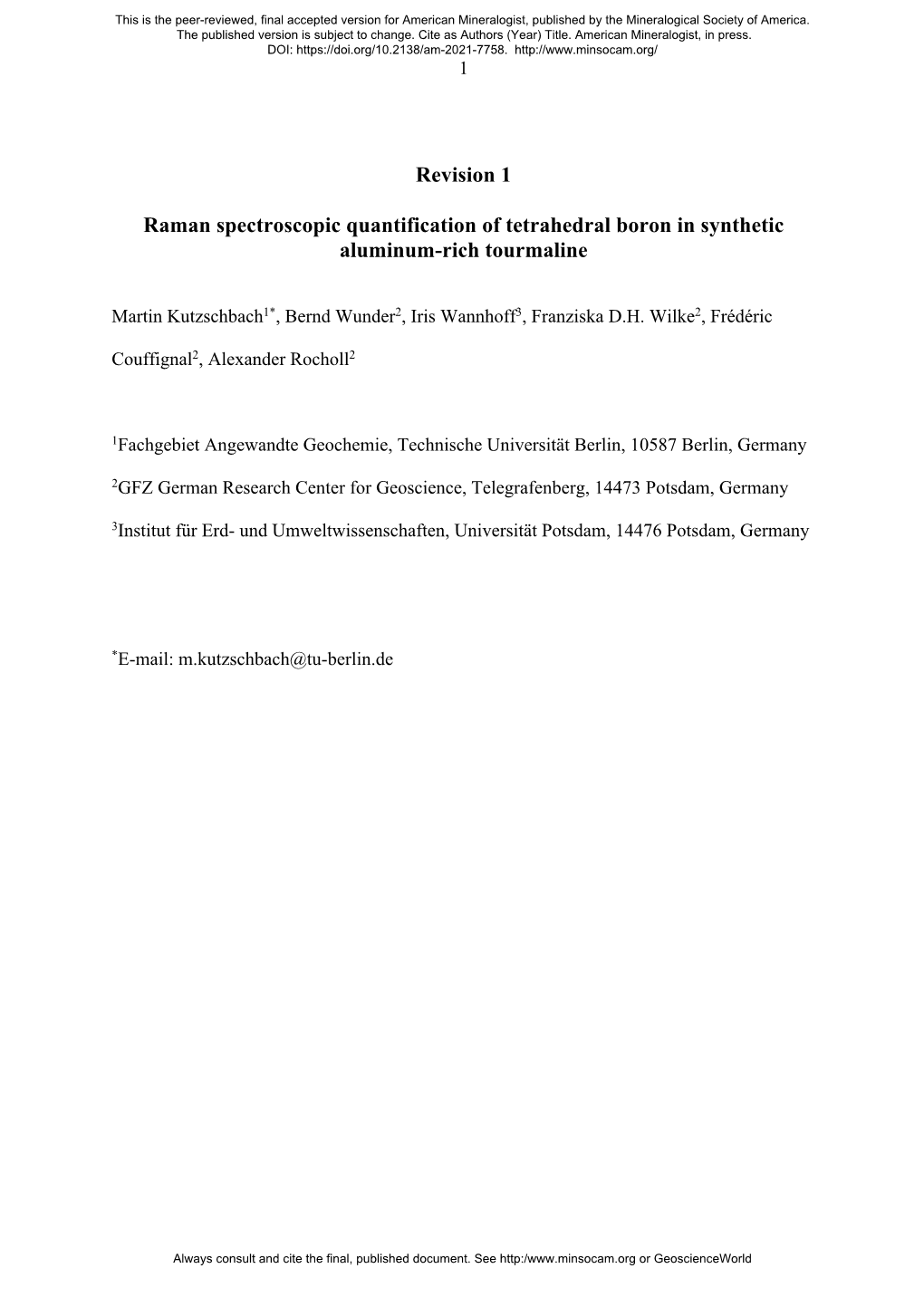
Load more
Recommended publications
-
Tourmaline Composition of the Kışladağ Porphyry Au Deposit, Western Turkey: Implication of Epithermal Overprint
minerals Article Tourmaline Composition of the Kı¸slada˘gPorphyry Au Deposit, Western Turkey: Implication of Epithermal Overprint Ömer Bozkaya 1,* , Ivan A. Baksheev 2, Nurullah Hanilçi 3, Gülcan Bozkaya 1, Vsevolod Y. Prokofiev 4 , Yücel Özta¸s 5 and David A. Banks 6 1 Department of Geological Engineering, Pamukkale University, 20070 Denizli, Turkey; [email protected] 2 Department of Geology, Moscow State University, Leninskie Gory, 119991 Moscow, Russia; [email protected] 3 Department of Geological Engineering, Istanbul University-Cerrahpa¸sa,Avcılar, 34320 Istanbul, Turkey; [email protected] 4 Institute of Geology of Ore Deposits, Petrography, Mineralogy and Geochemistry, Russian Academy of Sciences, 119017 Moscow, Russia; [email protected] 5 TÜPRAG Metal Madencilik, Ovacık Mevki Gümü¸skolKöyü, Ulubey Merkez, 64902 U¸sak,Turkey; [email protected] 6 School of Earth and Environment, University of Leeds, Leeds LS2 9JT, UK; [email protected] * Correspondence: [email protected]; Tel.: +90-258-296-3442 Received: 13 August 2020; Accepted: 4 September 2020; Published: 7 September 2020 Abstract: The Kı¸slada˘gporphyry Au deposit occurs in a middle Miocene magmatic complex comprising three different intrusions and magmatic-hydrothermal brecciation related to the multiphase effects of the different intrusions. Tourmaline occurrences are common throughout the deposit, mostly as an outer alteration rim around the veins with lesser amounts disseminated in the intrusions, and are associated with every phase of mineralization. Tourmaline mineralization has developed as a tourmaline-rich matrix in brecciated zones and tourmaline-quartz and/or tourmaline-sulfide veinlets within the different intrusive rocks. Tourmaline was identified in the tourmaline-bearing breccia zone (TBZ) and intrusive rocks that had undergone potassic, phyllic, and advanced argillic alteration. -
Magnesio-Lucchesiite, Camg3al6(Si6o18)(BO3)3(OH)3O, a New Species of the Tourmaline Supergroup
American Mineralogist, Volume 106, pages 862–871, 2021 Magnesio-lucchesiite, CaMg3Al6(Si6O18)(BO3)3(OH)3O, a new species of the tourmaline supergroup Emily D. Scribner1,2, Jan Cempírek3,*,†, Lee A. Groat2, R. James Evans2, Cristian Biagioni4, Ferdinando Bosi5,*, Andrea Dini6, Ulf Hålenius7, Paolo Orlandi4, and Marco Pasero4 1Environmental Engineering and Earth Sciences, Clemson University, 445 Brackett Hall, 321 Calhoun Drive, Clemson, South Carolina 29634, U.S.A. 2Department of Earth, Ocean and Atmospheric Sciences, University of British Columbia, Vancouver, British Columbia V6T 1Z4, Canada 3Department of Geological Sciences, Faculty of Science, Masaryk University, Brno, 611 37, Czech Republic 4Dipartimento di Scienze della Terra, Università di Pisa, Via Santa Maria 53, I-56126 Pisa, Italy 5Dipartimento di Scienze della Terra, Sapienza Università di Roma, Piazzale Aldo Moro 5, I-00185, Rome, Italy 6Istituto di Geoscienze e Georisorse-CNR, Via Moruzzi 1, 56124 Pisa, Italy 7Department of Geosciences, Swedish Museum of Natural History, P.O. Box 50 007, 104 05 Stockholm, Sweden Abstract Magnesio-lucchesiite, ideally CaMg3Al6(Si6O18)(BO3)3(OH)3O, is a new mineral species of the tourmaline supergroup. The holotype material was discovered within a lamprophyre dike that cross- cuts tourmaline-rich metapelites within the exocontact of the O’Grady Batholith, Northwest Territories (Canada). Two additional samples were found at San Piero in Campo, Elba Island, Tuscany (Italy) in hydrothermal veins embedded in meta-serpentinites within the contact aureole of the Monte Capanne intrusion. The studied crystals of magnesio-lucchesiite are black in a hand sample with vitreous luster, conchoidal fracture, an estimated hardness of 7–8, and a calculated density of 3.168 (Canada) and 3.175 g/cm3 (Italy). -
Gem-Quality Tourmaline from LCT Pegmatite in Adamello Massif, Central Southern Alps, Italy: an Investigation of Its Mineralogy, Crystallography and 3D Inclusions
minerals Article Gem-Quality Tourmaline from LCT Pegmatite in Adamello Massif, Central Southern Alps, Italy: An Investigation of Its Mineralogy, Crystallography and 3D Inclusions Valeria Diella 1,* , Federico Pezzotta 2, Rosangela Bocchio 3, Nicoletta Marinoni 1,3, Fernando Cámara 3 , Antonio Langone 4 , Ilaria Adamo 5 and Gabriele Lanzafame 6 1 National Research Council, Institute for Dynamics of Environmental Processes (IDPA), Section of Milan, 20133 Milan, Italy; [email protected] 2 Natural History Museum, 20121 Milan, Italy; [email protected] 3 Department of Earth Sciences “Ardito Desio”, University of Milan, 20133 Milan, Italy; [email protected] (R.B.); [email protected] (F.C.) 4 National Research Council, Institute of Geosciences and Earth Resources (IGG), Section of Pavia, 27100 Pavia, Italy; [email protected] 5 Italian Gemmological Institute (IGI), 20123 Milan, Italy; [email protected] 6 Elettra-Sincrotrone Trieste S.C.p.A., Basovizza, 34149 Trieste, Italy; [email protected] * Correspondence: [email protected]; Tel.: +39-02-50315621 Received: 12 November 2018; Accepted: 7 December 2018; Published: 13 December 2018 Abstract: In the early 2000s, an exceptional discovery of gem-quality multi-coloured tourmalines, hosted in Litium-Cesium-Tantalum (LCT) pegmatites, was made in the Adamello Massif, Italy. Gem-quality tourmalines had never been found before in the Alps, and this new pegmatitic deposit is of particular interest and worthy of a detailed characterization. We studied a suite of faceted samples by classical gemmological methods, and fragments were studied with Synchrotron X-ray computed micro-tomography, which evidenced the occurrence of inclusions, cracks and voids. -
Revision 2 Evidence of Permian Magmatism in the Alpi Apuane Metamorphic Complex
*Revised Manuscript with No changes marked Click here to download Revised Manuscript with No changes marked: Fornovolasco_Metarhyolite_Lithos_rev2.docx 1 Revision 2 2 Evidence of Permian magmatism in the Alpi Apuane Metamorphic Complex 3 (Northern Apennines, Italy): new hints for the geological evolution of the 4 basement of the Adria plate 5 6 Simone Vezzoni1, Cristian Biagioni1*, Massimo D’Orazio1, Diego Pieruccioni1, Yuri Galanti1, 7 Maurizio Petrelli2,3, and Giancarlo Molli1 8 9 1 Dipartimento di Scienze della Terra, Università di Pisa, Via S. Maria 53, I-56126, Pisa, Italy 10 2 Dipartimento di Fisica e Geologia, Università di Perugia, Piazza dell’Università, I-06123 11 Perugia, Italy 12 3.Istituto Nazionale di Fisica Nucleare, Sezione di Perugia, Via Alessandro Pascoli 23c, I-06123 13 Perugia, Italy 14 15 *Corresponding author: [email protected] 16 17 1. Introduction 18 19 The Paleozoic basement of the Northern Apennines (central-northern Italy) has been described 20 from few small outcrops, with the only exception being represented by the Alpi Apuane where large 21 outcrops (ca. 60 km2) of pre-Triassic formations are widely exposed. During the Alpine tectonic 22 evolution, these formations were part of the basement of the Adria plate which, according to 23 different authors (e.g., Stampfli and Borel, 2002; Stampfli et al., 2002; von Raumer et al., 2013), 24 was located, in the Paleozoic paleotectonic frame, along the northern margin of Gondwana, 25 therefore recording the tectono-sedimentary and magmatic processes associated with its 26 geodynamic evolution (e.g., Sirevaag et al., 2017). Indeed, during the late Neoproterozoic-middle 27 Paleozoic, the northern margin of Gondwana was modified first by crustal accretion and then by 28 repeated rifting and associated intense magmatism (e.g., Romeo et al., 2006; von Raumer et al., 29 2013). -
Using Tourmaline As an Indicator of Provenance
Louisiana State University LSU Digital Commons LSU Master's Theses Graduate School 2016 Using Tourmaline As An Indicator Of Provenance: Development And Application Of A Statistical Approach Using Random Forests Erin Lael Walden Louisiana State University and Agricultural and Mechanical College, [email protected] Follow this and additional works at: https://digitalcommons.lsu.edu/gradschool_theses Part of the Earth Sciences Commons Recommended Citation Walden, Erin Lael, "Using Tourmaline As An Indicator Of Provenance: Development And Application Of A Statistical Approach Using Random Forests" (2016). LSU Master's Theses. 4490. https://digitalcommons.lsu.edu/gradschool_theses/4490 This Thesis is brought to you for free and open access by the Graduate School at LSU Digital Commons. It has been accepted for inclusion in LSU Master's Theses by an authorized graduate school editor of LSU Digital Commons. For more information, please contact [email protected]. USING TOURMALINE AS AN INDICATOR OF PROVENANCE: DEVELOPMENT AND APPLICATION OF A STATISTICAL APPROACH USING RANDOM FORESTS A Thesis Submitted to the Graduate Faculty of the Louisiana State University and Agricultural and Mechanical College in partial fulfillment of the requirements of the degree of Master of Science in The Department of Geology and Geophysics by Erin Lael Walden B.S., Louisiana State University, 2008 December 2016 In memory of Wayne Douglas Walden ii ACKNOWLEDGMENTS My sincere and endless gratitude goes to Darrell Henry, who provided funding for two years and saw me through personal and academic hurdles with tireless patience and understanding. His insight and communication improved this manuscript greatly. I must also thank Barbara Dutrow for her suggestions and contributions, and Brian Marx for his expertise in statistics and help with programming. -
Celkový Seznam Publikací
Přehled impaktovaných publikací učitelů/studentů ÚGV PřF MU v Brně v období 2003-2021 2021 (celkem 7 článků, 3 studenti spoluautoři – červeně) Frýbort, A., Štulířová, J., Zavřel, T., Gregerová, M., Všianský, D. (2021): Reactivity of slag in 15 years old self-compacting concrete. Construction and Building Materials, 267, 120914. doi: 10.1016/j.conbuildmat.2020.120914 WoS: Gadas, P., Novák, M., Vašinová Galiová, M., Szuszkiewicz, A., Pieczka, A., Haifler, J., Cempírek, J. (2021): Secondary Beryl in Cordierite/Sekaninaite Pseudomorphs from Granitic Pegmatites – A Monitor of Elevated Content of Beryllium in the Precursor. Canadian Mineralogist. In press. doi: 10.3749/canmin.2000014 WoS: Haifler, J., Škoda, R., Filip, J., Larsen, A.O., Rohlíček, J. (2021): Zirconolite from Larvik Plutonic Complex, Norway, its relationship to stefanweissite and nöggerathite, and contgribution to the improvement of zirconolite endmember systematice. American Mineralogist. In press. doi: 10.2138/am-2021-7510 WoS: Krmíček, L., Novák, M., Trumbull, R.B., Cempírek, J., Houzar, S. (2021): Boron isotopic variations in tourmaline from metacarbonattes and associated talc-silicate rocks from the Bohemian Massif: Constraints on boron recycling in the Variscan orogen. Geoscience Frontiers, 12, 1, 219–230. doi: 10.1016/j.gsf.2020.03.009 WoS: Krmíček, L., Ulrych, J., Jelínek, E., Skála, R., Krmíčková, S., Korbelová, Z., Balogh, K. (2021): Petrogenesis of Cenozoic high-Mg (picritic) volcanic rocks in the České středohoří Mts. (Bohemian Massif, Czech Republic). Mineralogy and Petrology. In press. doi: 10.1007/s00710-020-00729-5 WoS: Majzlan, J., Plášil, J., Dachs, E., Benisek, A., Mangold, S., Škoda, R., Abrosimova, N. (2021): Prediction and observation of formation of Ca–Mg arsenates in acidic and alkaline fluids: Thermodynamic properties and mineral assemblages at Jáchymov, Czech Republic and Rotgülden, Austria. -
Lucchesiite Cafe 3Al6(Si6o18)(BO3)3(OH)3O
2+ Lucchesiite CaFe 3Al6(Si6O18)(BO3)3(OH)3O Crystal Data: Hexagonal. Point Group: 3m. Crystals prismatic, to 5 cm. Physical Properties: Cleavage: [Poor/indistinct on {0001}.] Fracture: Conchoidal. Tenacity: Brittle. Hardness = ~ 7 D(calc.) = 3.209 (Sri Lanka), 3.243 (Czech Republic) Optical Properties: Transparent. Color: Black. Streak: Gray. Luster: Vitreous. Optical Class: Uniaxial (-). ω = 1.670(5) ε = 1.655(5) Pleochroism: O = very dark brown, E = light brown. Cell Data: Space Group: R3m. a = 16.0018(7) c = 7.2149(3) Z = 3 X-ray Powder Pattern: Sri Lanka. 2.587 (100), 2.970 (99), 3.490 (72), 2.049 (69), 1.926 (43), 4.236 (42), 1.512 (42) Chemistry: (1) (2) (1) (2) SiO2 34.03 33.46 MnO 0.05 0.20 TiO2 2.53 0.64 ZnO 0.10 0.04 B2O3 [10.11] [9.89] CaO 3.74 2.62 Al2O3 26.48 27.00 Na2O 0.89 1.32 V2O3 0.12 b.d.l. K2O 0.09 0.06 FeOtotal 11.77 16.82 F 0.44 0.10 Fe2O3 [1.97] [10.05] H2O [2.67] [2.27] FeO [10.00] [7.77] –O = F2 0.19 0.04 MgO 6.73 3.59 Total 99.76 98.96 (1) Sri Lanka; average of 10 electron microprobe analyses supplemented by Mössbauer and FTIR X spectrometry, H2O, B2O3,Fe2O3 and FeO calculated; corresponds to (Ca0.69Na0.30K0.02)Σ=1.01 Y 2+ 4+ 3+ Z 3+ T (Fe 1.44Mg0.72Al0.48Ti 0.33V 0.02Mn0.01Zn0.01)Σ=3.00 (Al4.74Mg1.01Fe 0.25)Σ=6.00[ (Si5.85Al0.15)Σ=6.00O18] V W (BO3)3 (OH)3 [O0.69F0.24(OH)0.07]Σ=1.00. -
Tourmaline Crystal Chemistry
1 Revision 1 2 Tourmaline crystal chemistry 3 4 FERDINANDO BOSI 5 Department of Earth Sciences, Sapienza University di Rome, Piazzale Aldo Moro 5, 00185 Rome, Italy 6 7 8 ABSTRACT 9 Tourmalines form the most important boron rock-forming minerals on Earth. They belong to 10 the cyclosilicates with a structure that may be regarded as a three-dimensional framework of 11 octahedra ZO6 that encompass columns of structural “islands” made of XO9, YO6, BO3 and TO4 12 polyhedra. The overall structure of tourmaline is a result of short-range and long-range constraints 13 depending respectively on the charge and size of ions. In this study, published data are reviewed 14 and analyzed to achieve a synthesis of relevant experimental results and to construct a crystal- 15 chemical model for describing tourmalines and their compositional miscibility over different length 16 scales. Order-disorder substitution reactions involving cations and anions are controlled by short- 17 range structural constraints, whereas order-disorder intracrystalline reaction involving only cations 18 are controlled by long-range structural constraints. The chemical affinity of a certain cation to a 19 specific structural site of the tourmaline structure has been established on the basis of structural data 20 and crystal-chemical considerations. This has direct implications for the tourmaline nomenclature, 21 as well as on petrogenetic and provenance information. Some assumptions behind the classification 22 scheme of tourmaline have been reformulated, revealing major agreement and significant 23 improvements compared to earlier proposed scheme. 24 25 Keywords: Tourmaline, order-disorder, crystal structure, nomenclature 1 26 27 28 INTRODUCTION 29 The tourmaline supergroup minerals are chemically complex cyclosilicates rich in boron. -
6679 2 3 MAGNESIO-LUCCHESIITE, Camg 3Al6(Si6o18)(BO3)3(OH)3O
This is the peer-reviewed, final accepted version for American Mineralogist, published by the Mineralogical Society of America. The published version is subject to change. Cite as Authors (Year) Title. American Mineralogist, in press. DOI: https://doi.org/10.2138/am-2021-7496. http://www.minsocam.org/ 1 Revision 1 2 Word Count: 6679 3 4 MAGNESIO-LUCCHESIITE, CaMg3Al6(Si6O18)(BO3)3(OH)3O, A NEW SPECIES OF 5 THE TOURMALINE SUPERGROUP 6 7 EMILY D. SCRIBNER1,2, JAN CEMPÍREK3, * , LEE A. GROAT2, R. JAMES EVANS2, 8 CRISTIAN BIAGIONI4, FERDINANDO BOSI5,*, ANDREA DINI6, ULF HÅLENIUS7, 9 PAOLO ORLANDI4, MARCO PASERO4 10 11 1Environmental Engineering and Earth Sciences, Clemson University, 445 Brackett Hall, 321 12 Calhoun Drive, Clemson, South Carolina 29634, USA 13 2Department of Earth, Ocean and Atmospheric Sciences, University of British Columbia, 14 Vancouver, British Columbia V6T 1Z4, Canada 15 3Department of Geological Sciences, Faculty of Science, Masaryk University, Brno, CZ-65937, 16 Czech Republic 17 4Dipartimento di Scienze della Terra, Università di Pisa, Via Santa Maria 53, I-56126 Pisa, Italy 18 5Dipartimento di Scienze della Terra, Sapienza Università di Roma, Piazzale Aldo Moro 5, I- 19 00185, Rome, Italy 20 6Institute of Geosciences and Georisorse-CNR, Via Moruzzi 1, 56124 Pisa, Italy 21 7Department of Geosciences, Swedish Museum of Natural History, P.O. Box 50 007, 104 05 22 Stockholm, Sweden * E-mail address: [email protected]; [email protected] 1 Always consult and cite the final, published document. See http:/www.minsocam.org or GeoscienceWorld This is the peer-reviewed, final accepted version for American Mineralogist, published by the Mineralogical Society of America. -
IMA–CNMNC Approved Mineral Symbols
Mineralogical Magazine (2021), 85, 291–320 doi:10.1180/mgm.2021.43 Article IMA–CNMNC approved mineral symbols Laurence N. Warr* Institute of Geography and Geology, University of Greifswald, 17487 Greifswald, Germany Abstract Several text symbol lists for common rock-forming minerals have been published over the last 40 years, but no internationally agreed standard has yet been established. This contribution presents the first International Mineralogical Association (IMA) Commission on New Minerals, Nomenclature and Classification (CNMNC) approved collection of 5744 mineral name abbreviations by combining four methods of nomenclature based on the Kretz symbol approach. The collection incorporates 991 previously defined abbreviations for mineral groups and species and presents a further 4753 new symbols that cover all currently listed IMA minerals. Adopting IMA– CNMNC approved symbols is considered a necessary step in standardising abbreviations by employing a system compatible with that used for symbolising the chemical elements. Keywords: nomenclature, mineral names, symbols, abbreviations, groups, species, elements, IMA, CNMNC (Received 28 November 2020; accepted 14 May 2021; Accepted Manuscript published online: 18 May 2021; Associate Editor: Anthony R Kampf) Introduction used collection proposed by Whitney and Evans (2010). Despite the availability of recommended abbreviations for the commonly Using text symbols for abbreviating the scientific names of the studied mineral species, to date < 18% of mineral names recog- chemical elements -
High‐Temperature Fe Oxidation Coupled with Redistribution Of
research papers High-temperature Fe oxidation coupled with redistribution of framework cations in lobanovite, K Na(Fe2+ Mg Na)Ti (Si O ) O (OH) – the first ISSN 2052-5206 2 4 2 2 4 12 2 2 4 titanosilicate case Elena S. Zhitova,a* Andrey A. Zolotarev,a Frank C. Hawthorne,b a,c c a Received 19 February 2019 Sergey V. Krivovichev, Viktor N. Yakovenchuk and Alexey G. Goncharov Accepted 30 April 2019 aInstitute of Earth Sciences, St Petersburg State University, University Emb. 7/9, St Petersburg, 199034, Russian Federation, bDepartment of Geological Sciences, University of Manitoba, 125 Dysart Road (Wallace Building), Winnipeg, MB R3T Edited by R. Cˇ erny´, University of Geneva, 2N2, Canada, and cNanomaterials Research Center, Kola Science Center, Russian Academy of Sciences, Fersmana Str. 14, Switzerland Apatity, 184209, Russian Federation. *Correspondence e-mail: [email protected] Keywords: iron oxidation; dehydrogenation; 2+ The high-temperature (HT) behaviour of lobanovite, K2Na(Fe 4Mg2Na)Ti2- cation redistribution/migration; astrophyllite- in situ supergroup minerals; titanosilicate; hetero- (Si4O12)2O2(OH)4, was studied using powder X-ray diffraction in the phyllosilicate. temperature range 25–1000 C and ex situ single-crystal X-ray diffraction of 17 crystals quenched from different temperatures. HT iron oxidation associated CCDC references: 1889997; 1889998; with dehydroxylation starts at 450 C, similar to other ferrous-hydroxy-rich 1889999; 1890000; 1890001; 1890002; heterophyllosilicates such as astrophyllite and bafertisite. A prominent feature 1890003; 1890004; 1890005; 1890006; of lobanovite HT crystal chemistry is the redistribution of Fe and Mg+Mn 1890007; 1890008; 1890009; 1890010; M M M 1890011; 1890012; 1890013 cations over the (2), (3), (4) sites of the octahedral (O) layer that accompanies iron oxidation and dehydroxylation. -
Revision 1 of Manuscript #5820 Influence of the Octahedral Cationic
1 Revision 1 of manuscript #5820 2 3 Influence of the octahedral cationic-site occupancies on the framework 4 vibrations of Li-free tourmalines 5 6 Anke Watenphul1*, Jochen Schlüter2, Ferdinando Bosi3, Henrik Skogby4, Thomas Malcherek1, and Boriana 7 Mihailova1 8 9 10 1Fachbereich Geowissenschaften, Universität Hamburg, Grindelallee 48, D-20146 Hamburg, Germany 11 2Centrum für Naturkunde, Mineralogisches Museum, Universität Hamburg, Grindelallee 48, D-20146 Hamburg 12 3Dipartimento die Scienze della Terra, Sapienza Università di Roma, Piazzale Aldo Moro 5, I-00185 Rome, Italy 13 4Department of Geosciences, Swedish Museum of Natural History, Box 50007, SE-10405 Stockholm, Sweden 14 15 16 *corresponding author: [email protected] 17 18 19 20 21 22 23 24 ABSTRACT 25 Tourmalines, XY3Z6T6O18(BO3)3V3W, are excellent petrogenetic indicators as they capture the 26 signature of the host rock bulk composition. Raman spectra of tourmalines can be used as fingerprints for species 27 identification and crystal chemical analysis. While Li-bearing species are directly distinguishable by the shape of 28 the OH-stretching vibrations, the discrimination of Mg- and Fe-dominant species can be hindered by the 29 coexistence of at least 2 types of octahedrally coordinated Rn+ cations. 30 Thirty Li-free tourmaline samples comprising fourteen different species were studied by Raman 31 spectroscopy and electron microprobe. All nine Fe3+-bearing samples were analyzed also by single-crystal X-ray 32 diffraction and Mössbauer spectroscopy. The Raman scattering analysis shows that Mg-dominant species can be 33 immediately distinguished from Fe-dominant species by the shape of the vibrational modes at ~200-240 cm-1 34 arising from the YO6 vibrations.