Tsunami Hazard Modelling Guidelines AUSTRALIAN DISASTER RESILIENCE HANDBOOK COLLECTION Tsunami Hazard Modelling Guidelines
Total Page:16
File Type:pdf, Size:1020Kb
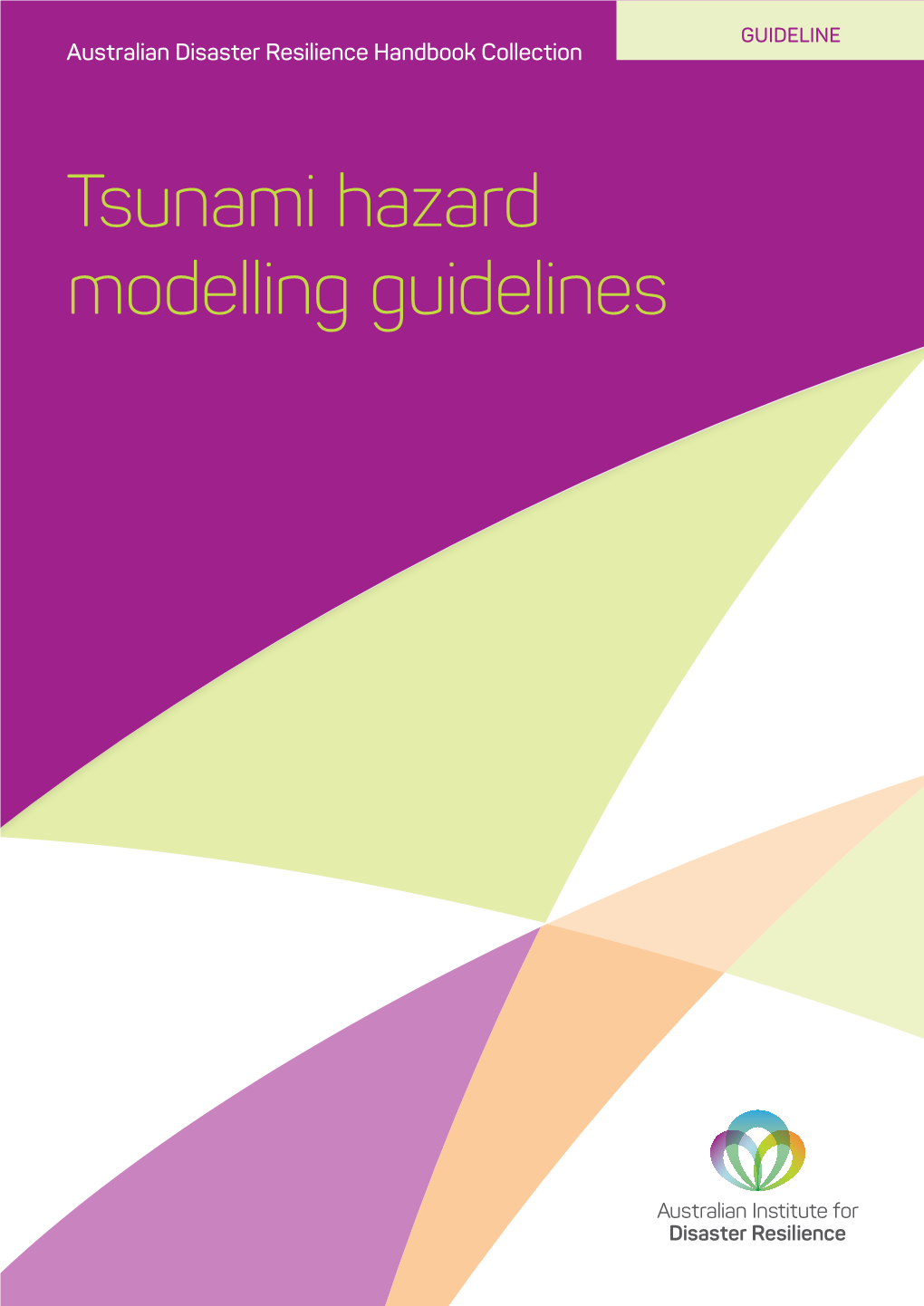
Load more
Recommended publications
-
Understanding Eddy Field in the Arctic Ocean from High-Resolution Satellite Observations Igor Kozlov1, A
Abstract ID: 21849 Understanding eddy field in the Arctic Ocean from high-resolution satellite observations Igor Kozlov1, A. Artamonova1, L. Petrenko1, E. Plotnikov1, G. Manucharyan2, A. Kubryakov1 1Marine Hydrophysical Institute of RAS, Russia; 2School of Oceanography, University of Washington, USA Highlights: - Eddies are ubiquitous in the Arctic Ocean even in the presence of sea ice; - Eddies range in size between 0.5 and 100 km and their orbital velocities can reach up to 0.75 m/s. - High-resolution SAR data resolve complex MIZ dynamics down to submesoscales [O(1 km)]. Fig 1. Eddy detection Fig 2. Spatial statistics Fig 3. Dynamics EGU2020 OS1.11: Changes in the Arctic Ocean, sea ice and subarctic seas systems: Observations, Models and Perspectives 1 Abstract ID: 21849 Motivation • The Arctic Ocean is a host to major ocean circulation systems, many of which generate eddies transporting water masses and tracers over long distances from their formation sites. • Comprehensive observations of eddy characteristics are currently not available and are limited to spatially and temporally sparse in situ observations. • Relatively small Rossby radii of just 2-10 km in the Arctic Ocean (Nurser and Bacon, 2014) also mean that most of the state-of-art hydrodynamic models are not eddy-resolving • The aim of this study is therefore to fill existing gaps in eddy observations in the Arctic Ocean. • To address it, we use high-resolution spaceborne SAR measurements to detect eddies over the ice-free regions and in the marginal ice zones (MIZ). EGU20 -OS1.11 – Kozlov et al., Understanding eddy field in the Arctic Ocean from high-resolution satellite observations 2 Abstract ID: 21849 Methods • We use multi-mission high-resolution spaceborne synthetic aperture radar (SAR) data to detect eddies over open ocean and marginal ice zones (MIZ) of Fram Strait and Beaufort Gyre regions. -
The General Circulation of the Atmosphere and Climate Change
12.812: THE GENERAL CIRCULATION OF THE ATMOSPHERE AND CLIMATE CHANGE Paul O'Gorman April 9, 2010 Contents 1 Introduction 7 1.1 Lorenz's view . 7 1.2 The general circulation: 1735 (Hadley) . 7 1.3 The general circulation: 1857 (Thompson) . 7 1.4 The general circulation: 1980-2001 (ERA40) . 7 1.5 The general circulation: recent trends (1980-2005) . 8 1.6 Course aim . 8 2 Some mathematical machinery 9 2.1 Transient and Stationary eddies . 9 2.2 The Dynamical Equations . 12 2.2.1 Coordinates . 12 2.2.2 Continuity equation . 12 2.2.3 Momentum equations (in p coordinates) . 13 2.2.4 Thermodynamic equation . 14 2.2.5 Water vapor . 14 1 Contents 3 Observed mean state of the atmosphere 15 3.1 Mass . 15 3.1.1 Geopotential height at 1000 hPa . 15 3.1.2 Zonal mean SLP . 17 3.1.3 Seasonal cycle of mass . 17 3.2 Thermal structure . 18 3.2.1 Insolation: daily-mean and TOA . 18 3.2.2 Surface air temperature . 18 3.2.3 Latitude-σ plots of temperature . 18 3.2.4 Potential temperature . 21 3.2.5 Static stability . 21 3.2.6 Effects of moisture . 24 3.2.7 Moist static stability . 24 3.2.8 Meridional temperature gradient . 25 3.2.9 Temperature variability . 26 3.2.10 Theories for the thermal structure . 26 3.3 Mean state of the circulation . 26 3.3.1 Surface winds and geopotential height . 27 3.3.2 Upper-level flow . 27 3.3.3 200 hPa u (CDC) . -
Metocean Design Condition for “Fukushima Forward” Project
Grand Renewable Energy 2014, July27-August 1 O-WdOc-1-4 METOCEAN DESIGN CONDITION FOR “FUKUSHIMA FORWARD” PROJECT Takeshi Ishihara 1, Kenji Shimada 2 and Akihiko Imakita 3 1 The University of Tokyo, 7-3-1 Hongo, Bunkyo-ku, 113-8656 Japan 2 Shimizu Corporation, 3-4-17 Etchujima, Koto-ku, Tokyo, 135-8530 Japan 3 Mitsui Engineering & Shipbuilding, 5-6-4 Tsukiji, Chuo-ku, Tokyo, 104-8439 Japan Metocean condition for the Fukushima FORWARD project is presented. Wind speeds and tsunami are predicted by Monte Carlo simulation and Tsunami simulation, respectively. For wave condition, extreme sea state and normal sea state are evaluated by published data and newly proposed wind-wave and swell combination formula, respectively. Surface current and water level are evaluated by extreme value analyses of hindcast simulation and historical data, respectively. Keywords: floating wind turbine, design conditions, Monte Carlo simulation, extreme value, tsunami INTRODUCTION the extratropical cyclones when estimating extreme wind. Therefore, in this study, 50 year extreme wind speed was For the revitalization in Fukushima prefecture from evaluated by synthesizing probabilities of disasters by the Tohoku region Pacific coast earthquake non-exceedance of two independent processes, i.e. and accident of Fukushima Daiichi nuclear power plant in typhoon FT uand extratropical cyclone FE u by Eq.(1) 2011, Japanese government initiated the world first [1]. Figure 2 shows the synthesis of the probability Floating OffshRe Wind fARm Demonstration project distributions of annual maximum wind speeds by tropical (FORWARD project). In this paper, results of assessment and extratropical cyclone, where probabilities of on metocean conditions for 2MW floating wind turbine and non-exceedance of the annual maximum wind speed was the world first floating substation are presented for wind calculated by Monte Carlo simulation of 10000 years for speed, water level, wave, current and tsunami. -
DE-004 Metocean Engineering and Oceanography Fundamentals
Subsea Engineering Competency Profile METOCEAN ENGINEERING AND OCEANOGRAPHY FUNDAMENTALS DE-004 This competency demonstrates a subsea engineer has a broad understanding of metocean engineering and its application to subsea engineering. WHAT THIS COMPETENCE MEANS IN ELEMENT OF COMPETENCE INDICATORS OF ATTAINMENT PRACTICE Working knowledge of how metocean parameters are Requires that metocean parameters are analysed and Has used metocean criteria in subsea engineering applied in subsea engineering for: presented in a manner which is fit for engineering end analysis or design use ● design Has communicated interpretation of metocean ● installation information to others for subsea engineering application. ● operations (monitoring, fatigue, etc.) Working knowledge of operational and tropical cyclone Ability to use forecasts when following Offshore Demonstrated ability to interpret weather forecasts for weather forecast products available. Procedures and Cyclone Response Plans. safe operations offshore, and for cyclone avoidance. Working knowledge of the elements of subsea Ensures metocean risks are defined for subsea Has specified the requirements of metocean data engineering which require metocean input, the required engineering design elements. acquisition programmes, accounting for uncertainties in levels of accuracy and source be it regional, field the metocean site conditions, to satisfy subsea Ensures that metocean data acquisition is available in measured or modelled data engineering requirements on more than one project time at the required level of detail to reduce risks to phase. ALARP. Awareness of physical oceanography and marine Can explain regional conditions (e.g. cyclones, tides, Has worked with metocean engineers or consultants to meteorology including: eddies, solitons etc) and how these processes are define schedule and / or scope of metocean data likely to impact on site specific subsea design elements acquisition, modelling and studies for subsea ● winds including surface facilities behaviours. -
The Kingdom of Tonga Devastated by a Megatsunami in the Mid-15Th Century
EGU21-5317, updated on 25 Sep 2021 https://doi.org/10.5194/egusphere-egu21-5317 EGU General Assembly 2021 © Author(s) 2021. This work is distributed under the Creative Commons Attribution 4.0 License. The kingdom of Tonga devastated by a megatsunami in the mid-15th century Franck Lavigne1,2,3, Julie Morin4, Wassmer Patrick2, Weller Olivier5, Kula Taaniela6, Ana V. Maea6, Karim Kelfoun7, Fatima Mokadem2, Raphael Paris7, Mukhamad N. Malawani1,2,8, Faral Audrey1,2, Mhammed Benbakkar7, Ségolène Saulnier-Copard2, Céline M. Vidal4, Tu’I’ahai Tu’I’afitu6, Gomez Christopher9, and Fuka Kitekei’aho10 1Paris 1 Pantheon-Sorbonne University, ([email protected]) 2Laboratory of Physical Geography, UMR 8591 CNRS, Meudon, France 3Institut Universitaire de France, Paris, France 4Department of Geography, University of Cambridge, Cambridge, UK 5Trajectoires, UMR 8215 CNRS, Paris, France 6Ministry of Land and Natural Resources, Natural Resources Division, Nuku'alofa, Kingdom of Tonga 7Laboratoire Magmas et Volcans, Université Clermont Auvergne, CNRS, IRD, OPGC, France 8Faculty of Geography, Universitas Gadjah Mada, Yogyakarta, Indonesia 9Laboratory of Sediment Hazards and Disaster Risk, Kobe University, Japan 10Geocare & Petroleum Consult Ltd, Nuku'alofa, Kingdom of Tonga The pre-colonial history of Tonga and West Polynesia still suffers from major gaps because its reconstruction is essentially based on legends left by oral tradition, and by archaeological evidence somehow difficult to interpret. By the fourteenth century, the powerful Tu'i Tonga kingdom united the islands of the Tongan archipelago under a centralised authority and, according to tradition, extended its influence to neighbouring island groups in the Central Pacific. However, some periods of deep crisis were identified, e.g. -
Literature Review of Tsunami Sources Affecting Tsunami Hazard Along the US East Coast
Literature review of tsunami sources affecting tsunami hazard along the US East Coast Department of Ocean Engineering, University of Rhode Island Narragansett, USA Feb. 21, 2011 1 TABLE OF CONTENTS 1. BACKGROUND ...................................................................................................................... 3 2. LITERATURE REVIEW OF RELEVANT TSUNAMI SOURCES .................................... 5 2.1 Submarine Mass Failures ........................................................................................................ 5 2.2 Co-seismic tsunamis .................................................................................................................. 8 2.2.1 Review of literature on Caribbean subduction zone ............................................................. 8 2.2.2 NOAA Forecast Source Database for Caribbean subduction zone ............................... 16 2.2.3 Azores-Gibraltar convergence zone .......................................................................................... 17 2.3 Cumbre Vieja Volcano flanK collapse ............................................................................... 21 3. INITIAL SOURCE DEFINITIONS AND TSUNAMI SIMULATIONS .......................... 24 3.1 Modeling methodology .......................................................................................................... 24 3.1.1 Initial conditions for model .......................................................................................................... 24 3.1.2 Co-seismic sources -
Appendix E Metocean Report
VOWTAP Research Activities Plan Appendix E – Metocean Report October 2014 DOMINION RESOURCES SERVICES, INC METOCEAN CRITERIA FOR VOWTAP PROJECT OFFSHORE VIRGINIA METOCEAN CRITERIA FOR VIRGINIA OFFSHORE WIND TECHNOLOGY ADVANCEMENT PROJECT (VOWTAP) Report Number: C56462/7907/R3 Issue Date: 28 August 2013 This report is not to be used for contractual or engineering purposes unless described as ‘Final’ in the approval box below Prepared for: Dominion Resources Services, Inc Alternative Energy Solutions 3 Final Manuel Medina Wang Wensu Wang Wensu 28 August 2013 2 Updated Report Manuel Medina Shejun Fan Shejun Fan 09 August 2013 1 Updated Draft Report Manuel Medina Shejun Fan Shejun Fan 31 July 2013 Manuel Medina 0 Draft Report Shejun Fan Shejun Fan 17 July 2013 Jon Molina Rev Description Prepared Checked Approved Date Fugro GEOS/C56462/7907/R3 Page i DOMINION RESOURCES SERVICES, INC METOCEAN CRITERIA FOR VOWTAP PROJECT OFFSHORE VIRGINIA CONTENTS Page 1 INTRODUCTION 1 1.1 Units and Conventions 2 1.2 Abbreviations 2 1.3 Parameter Descriptions 3 2 METOCEAN CRITERIA 4 2.1 Wind Criteria 4 2.1.1 Omni-Directional 1-Year Extreme Wind Values 4 2.1.2 Directional 1-Year Extreme Wind Values 4 2.1.3 1-Year Wind Fitting Parameters 4 2.1.4 Omni-Directional Winter Storm Extreme Wind Values 5 2.1.5 Directional Winter Storm Extreme Wind Values 5 2.1.6 Omni-Directional Winter Storm Extreme Wind Values at Hub Height 6 2.1.7 Directional Winter Storm Extreme Wind Values at Hub Height 7 2.1.8 Wind Fitting Parameters for Winter Storm 8 2.1.9 Omni-Directional Hurricane -
Bildnachweis
Bildnachweis Im Bildnachweis verwendete Abkürzungen: With permission from the Geological Society of Ame- rica l – links; m – Mitte; o – oben; r – rechts; u – unten 4.65; 6.52; 6.183; 8.7 Bilder ohne Nachweisangaben stammen vom Autor. Die Autoren der Bildquellen werden in den Bildunterschriften With permission from the Society for Sedimentary genannt; die bibliographischen Angaben sind in der Literaturlis- Geology (SEPM) te aufgeführt. Viele Autoren/Autorinnen und Verlage/Institutio- 6.2ul; 6.14; 6.16 nen haben ihre Einwilligung zur Reproduktion von Abbildungen gegeben. Dafür sei hier herzlich gedankt. Für die nachfolgend With permission from the American Association for aufgeführten Abbildungen haben ihre Zustimmung gegeben: the Advancement of Science (AAAS) Box Eisbohrkerne Dr; 2.8l; 2.8r; 2.13u; 2.29; 2.38l; Box Die With permission from Elsevier Hockey-Stick-Diskussion B; 4.65l; 4.53; 4.88mr; Box Tuning 2.64; 3.5; 4.6; 4.9; 4.16l; 4.22ol; 4.23; 4.40o; 4.40u; 4.50; E; 5.21l; 5.49; 5.57; 5.58u; 5.61; 5.64l; 5.64r; 5.68; 5.86; 4.70ul; 4.70ur; 4.86; 4.88ul; Box Tuning A; 4.95; 4.96; 4.97; 5.99; 5.100l; 5.100r; 5.118; 5.119; 5.123; 5.125; 5.141; 5.158r; 4.98; 5.12; 5.14r; 5.23ol; 5.24l; 5.24r; 5.25; 5.54r; 5.55; 5.56; 5.167l; 5.167r; 5.177m; 5.177u; 5.180; 6.43r; 6.86; 6.99l; 6.99r; 5.65; 5.67; 5.70; 5.71o; 5.71ul; 5.71um; 5.72; 5.73; 5.77l; 5.79o; 6.144; 6.145; 6.148; 6.149; 6.160; 6.162; 7.18; 7.19u; 7.38; 5.80; 5.82; 5.88; 5.94; 5.94ul; 5.95; 5.108l; 5.111l; 5.116; 5.117; 7.40ur; 8.19; 9.9; 9.16; 9.17; 10.8 5.126; 5.128u; 5.147o; 5.147u; -
LONG WAVE SURGE Understanding Infragravity Wave Energy in Ports
LONG WAVE SURGE Understanding infragravity wave energy in ports Peter McComb Long period waves and harbour surges § What is the problem? § What is causing it? § What can we do about it? www.metocean.co.nz The problem 6 degrees of freedom www.metocean.co.nzwww.metocean.co.nz Long period waves (LPW) Red = inside harbour Blue = outside harbour Water level oscillations with periods of greater than swell but less than tides. Typically 25 – 1200 seconds with small amplitude. seiche § Bound long waves – tied to the wave group structure but can be released at the coast § Free long waves § Surf beat – long wave energy released in the surf zone FIG IG swell sea long waves www.metocean.co.nz Discovering LPW Infra-gravity 25-120s Total Far Infra-gravity 120-150+s Tide removed Wave group modulation (sets) Sea / Swell removed IG – often modulated by tide FIG – not modulated by tide www.metocean.co.nz Wave groups L h 1 1 2 E p = rgz.dz.dx = rgH Energy flux L òò 16 0 0 1 2 Et = E p + Ek = rgH 1 L h 1 1 8 E = r w2 + u 2 .dz.dx = rgH 2 k ò ò ( ) L 0 -h 2 16 www.metocean.co.nz IG and FIG waves Infra-gravity 25-120s IG Far Infra-gravity 120-150+s FIG 0.1 0.1 0.08 0.09 0.06 0.08 0.04 0.07 FIG waves are created by the modulation of wave energy 0.02 0.06 0 0.05 -0.02 0.04 into ‘sets’, which is beneficial for surfing. -
Physical Oceanography and Circulation in the Gulf of Mexico
Physical Oceanography and Circulation in the Gulf of Mexico Ruoying He Dept. of Marine, Earth & Atmospheric Sciences Ocean Carbon and Biogeochemistry Scoping Workshop on Terrestrial and Coastal Carbon Fluxes in the Gulf of Mexico St. Petersburg, FL May 6-8, 2008 Adopted from Oey et al. (2005) Adopted from Morey et al. (2005) Averaged field of wind stress for the GOM. [adapted from Gutierrez de Velasco and Winant, 1996] Surface wind Monthly variability Spring transition vs Fall Transition Adopted from Morey et al. (2005) Outline 1. General circulation in the GOM 1.1. The loop current and Eddy Shedding 1.2. Upstream conditions 1.3. Anticyclonic flow in the central and northwestern Gulf 1.4. Cyclonic flow in the Bay of Campeche 1.5. Deep circulation in the Gulf 2. Coastal circulation 2.1. Coastal Circulation in the Eastern Gulf 2.2. Coastal Circulation in the Northern Gulf 2.3. Coastal Circulation in the Western Gulf 1. General Circulation in the GOM 1.1. The Loop Current (LC) and Eddy Shedding Eddy LC Summary statistics for the Loop Current metrics computed from the January1, 1993 through July 1, 2004 altimetric time series Leben (2005) A compilation of the 31-yr Record (July 1973 – June 2004) of LC separation event. The separation intervals vary From a few weeks up to ~ 18 Months. Separation intervals tend to Cluster near 4.5-7 and 11.5, And 17-18.5 months, perhaps Suggesting the possibility of ~ a 6 month duration between each cluster. Leben (2005); Schmitz et al. (2005) Sturges and Leben (1999) The question of why the LC and the shedding process behave in such a semi-erratic manner is a bit of a mystery • Hurlburt and Thompson (1980) found erratic eddy shedding intervals in the lowest eddy viscosity run in a sequence of numerical experiment. -
Tsunamis Following the 1992 Nicaragua and Flores Events
Pure Appl. Geophys. 176 (2019), 2771–2793 Ó 2019 Springer Nature Switzerland AG https://doi.org/10.1007/s00024-019-02244-x Pure and Applied Geophysics Twenty-Five Years of Progress in the Science of ‘‘Geological’’ Tsunamis Following the 1992 Nicaragua and Flores Events 1 EMILE A. OKAL Abstract—We review a set of 47 tsunamis of geological origin Mindanao, Philippines, and in 1983 in the Sea of (triggered by earthquakes, landslides or volcanoes) which have Japan. While substantial progress was made in the occurred over the past 25 years and provided significant new insight into theoretical, experimental, field, or societal aspects of 1970s and 1980s on the theoretical and experimental tsunami science. Among the principal developments in our com- front (e.g., Hammack 1973; Ward 1980; Bernard and mand of various aspects of tsunamis, we earmark the development Milburn 1985), scientists still lacked the motivation of the W-phase inversion for the low-frequency moment tensor of the parent earthquake; the abandonment of the concept of a max- provided by exceptional and intriguing field imum earthquake magnitude for a given subduction zone, observations. controlled by simple plate properties; the development and The two tsunamis of 02 September 1992 in implementation of computer codes simulating the interaction of tsunamis with initially dry land at beaches, thus introducing a Nicaragua and 12 December 1992 in Flores Island, quantitative component to realistic tsunami warning procedures; Indonesia provided our community with a wealth of and the recent in situ investigation of current velocities, in addition challenges which spawned a large number of new to the field of surface displacements, during the interaction of investigations covering the observational, experi- tsunamis with harbors. -
Vaisala Met-Ocean System Components / for CRITICAL WEATHER CONDITIONS
Vaisala Met-Ocean System Components / FOR CRITICAL WEATHER CONDITIONS WEA-MAR-G-METOCEAN-brochure-B211367EN-B-210x280.indd 1 3.6.2014 13.20 World-Class Vaisala Sensor Technology Vaisala manufactures the widest selection of original meteorological sensors, systems and displays. Ultrasonic wind sensors • Vaisala's unique redundant triangle path technology always ensures turbulent-free measurement • Robust design enables functioning in rough conditions • DNV-approval makes Wind Sensor WMT700 an ideal choice for maritime environments • Body heating available for harsh offshore weather conditions • The FAA relies on Vaisala WINDCAP® technology Benefits ▪ The widest selection of original meteorological sensors and systems based on nearly 80 years of experience ▪ Extensive track record and global presence ▪ Easy upgrade of existing WMS to CAP437 compatible HMS ▪ Industry standard, approved by major oil companies ▪ All sensors are factory Barometric pressure All-in-one weather calibrated or tested and transmitters transmitter delivered with calibration certificate or factory test • Vaisala BAROCAP technology • WXT520 measures wind speed report ensures excellent long-term stability and direction, liquid precipitation, Vaisala systems have interfaces in various atmospheric pressure barometric pressure, temperature ▪ to wide range of sensors from measurements, even in outer space and relative humidity selected partners • Up to three pressure sensors for • Compact and durable with easy ▪ Field proven fully automatic redundancy in critical applications