A Two-Step Strategy for the Selective Conversion of Ethanol to Propene and Hydrogen
Total Page:16
File Type:pdf, Size:1020Kb
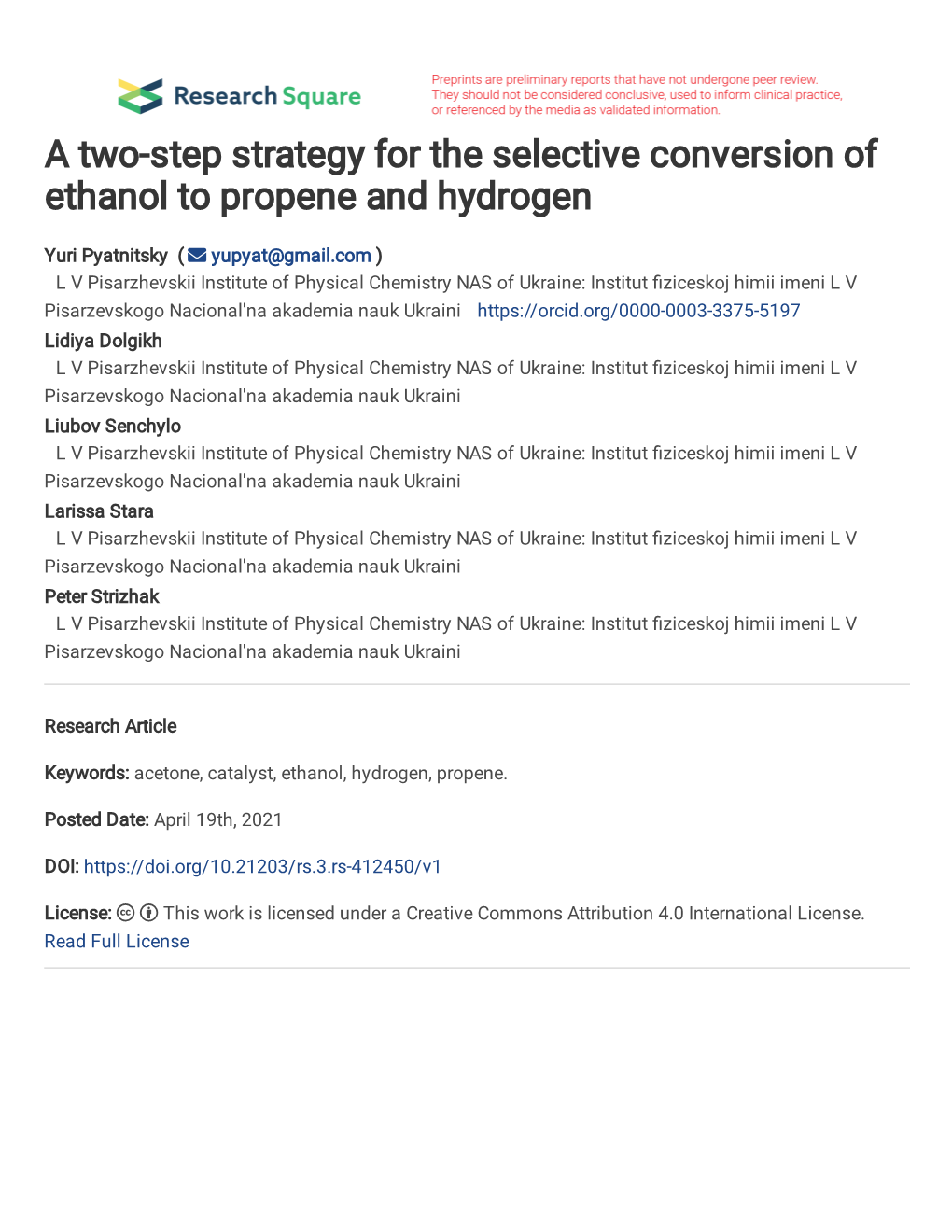
Load more
Recommended publications
-
Removal of NO and SO2 by Pulsed Corona Discharge Process
Korean J. Chem. Eng., 18(3), 308-316 (2001) Removal of NO and SO2 by Pulsed Corona Discharge Process Young Sun Mok†, Ho Won Lee, Young Jin Hyun, Sung Won Ham*, Jae Hak Kim** and In-Sik Nam** Department of Chemical Engineering, Cheju National University, Ara, Cheju 690-756, Korea *Department of Chemical Engineering, Kyungil University, Hayang, Kyungbuk 712-701, Korea **Department of Chemical Engineering, Pohang University of Science & Technology, Pohang, Kyungbuk 790-784, Korea (Received 6 September 2000 • accepted 9 March 2001) − Abstract Overall examination was made on the removal of NO and SO2 by pulsed corona discharge process. The mechanism for the removal of NO was found to largely depend on the gas composition. In the absence of oxygen, most of the NO removed was reduced to N2; on the other hand, oxidation of NO to NO2 was dominant in the presence of oxygen even when the content was low. Water vapor was an important ingredient for the oxidation of NO2 to nitric acid rather than that of NO to NO2. The removal of NO only slightly increased with the concentration of ammonia while the effect of ammonia on the removal of SO2 was very significant. The energy density (power delivered/feed gas flow rate) can be a measure for the degree of removal of NO. Regardless of the applied voltage and the flow rate of the feed gas stream, the amount of NO removed was identical at the same energy density. The production of N2O increased with the pulse repetition rate, and the presence of NH3 and SO2 enhanced it. -
Sds – Safety Data Sheet
Effective Date: 02/05/16 Replaces Revision: 01/01/13, 02/26/09 NON-EMERGENCY TELEPHONE 24-HOUR CHEMTREC EMERGENCY TELEPHONE 610-866-4225 800-424-9300 SDS – SAFETY DATA SHEET 1. Identification Product Identifier: ACETONE / ISOPROPYL ALCOHOL BLEND Synonyms: None Chemical Formula: Not Applicable to mixtures Recommended Use of the Chemical and Restrictions On Use: Laboratory Reagent Manufacturer / Supplier: Puritan Products; 2290 Avenue A, Bethlehem, PA 18017 Phone: 610-866-4225 Emergency Phone Number: 24-Hour Chemtrec Emergency Telephone 800-424-9300 2. Hazard(s) Identification Classification of the Substance or Mixture: Flammable liquids (Category 2) Skin irritation (Category 3) Eye irritation (Category 2A) Specific target organ toxicity - single exposure (Category 3) Risk Phrases: R11: Highly flammable. R36: Irritating to eyes. R66: Repeated exposure may cause skin dryness or cracking. R67: Vapors may cause drowsiness and dizziness. Label Elements: Trade Name: ACETONE / ISOPROPYL ALCOHOL BLEND Signal Word: Danger Hazard Statements: H225: Highly flammable liquid and vapor. H316: Causes mild skin irritation. H319: Causes serious eye irritation. H336: May cause drowsiness or dizziness. ACETONE / ISOPROPYL ALCOHOL BLEND Page 1 of 6 Precautionary Statements: P210: Keep away from heat/sparks/open flames/hot surfaces. No smoking. P261: Avoid breathing dust / fume / gas / mist / vapors / spray. P305 + P351 + P338: IF IN EYES: Rinse cautiously with water for several minutes. Remove contact lenses, if present and easy to do. Continue rinsing. 3. Composition / Information on Ingredients CAS Number: Not Applicable to mixtures Molecular Weight: Not Applicable to mixtures Ingredient CAS Number EC Number Percent Hazardous Chemical Characterization Acetone 67 - 64 - 1 200-662-2 70 - 90% Yes Substance Isopropyl Alcohol 67 - 63 - 0 200-661-7 10 - 30% Yes Substance 4. -
INF.20 Economic Commission for Europe Inland Transport Committee Definition Of
INF.20 Economic Commission for Europe Inland Transport Committee Working Party on the Transport of Dangerous Goods 6 March 2012 Joint Meeting of the RID Committee of Experts and the Working Party on the Transport of Dangerous Goods Bern, 19–23 March 2012 Item 5(a) of the provisional agenda Proposals for amendments to RID/ADR/ADN: pending issues Definition of LPG Transmitted by the Government of Switzerland We have observed contradictions in the definition of LPG adopted by the Joint Meeting at its Autumn 2010 session (ECE/TRANS/WP.15/AC.1/120, Annex II) as well as in the German translation we have discussed in Erfurt during the Conference of translation and edition of the German ADR. The actual definitions in each language are the following: ""Gaz de pétrole liquéfié (GPL)", un gaz liquéfié à faible pression contenant un ou plusieurs hydrocarbures légers qui sont affectés aux numéros ONU 1011, 1075, 1965, 1969 ou 1978 seulement et qui est principalement constitué de propane, de propène, de butane, des isomères du butane, de butène avec des traces d’autres gaz d’hydrocarbures. “‘Liquefied Petroleum Gas (LPG)’ means a low pressure liquefied gas composed of one or more light hydrocarbons which are assigned to UN Nos. 1011, 1075, 1965, 1969 or 1978 only and which consists mainly of propane, propene, butane, butane isomers, butene with traces of other hydrocarbon gases. „Flüssiggas (LPG)*: Unter geringem Druck verflüssigtes Gas, das aus einem oder mehreren leichten Kohlenwasserstoffen besteht, die nur der UN-Nummer 1011, 1075, 1965, 1969 oder 1978 zugeordnet sind und das hauptsächlich aus Propan, Propen, Butan, Butan-Isomeren und Buten mit Spuren anderer Kohlenwasserstoffgase besteht.“ One important point is different between French from one side and English and German from the other side, that is that in French the repetition of the “de” in the enumeration of possible gases brings to the conclusion that the main constituent of the LPG is one of the listed gases. -
SAFETY DATA SHEET Acetone
SAFETY DATA SHEET Acetone Revision date: 12.05.15 SDS/004/8 Page 1 of 6 1. Identification of the substance/mixture and of the company/undertaking 1.1. Product identifier Acetone EC No. 200-662-2 1.2. Relevant identified uses of the substance or mixture and uses advised against Household Solvent 1.3. Details of the supplier of the safety data sheet Thornton & Ross Ltd, Linthwaite, Huddersfield, HD7 5QH Tel: 01484 842217 Fax: 01484 847301 Email: [email protected] 1.4 Emergency telephone number: Out of normal working hours: +44 870 8510207 2. Hazards identification 2.1. Classification of the substance or mixture According to Regulation EC 1272/2008 classified as Flammable Liquid Category 2, Eye Irritant Category 2, Specific Target Organ Toxicity Single Exposure Category 3. 2.2. Label element GHS Pictogram Signal Word Hazard Class Danger Flammable Liquids, Category 2 Eye Irritation, Category 2 Specific Target Organ Toxicity-Single Exposure, Category 3 Hazard Statements Precautionary statements H225: Highly flammable liquid and vapour. P210: Keep away from heat/sparks/open flames/hot surfaces – No smoking. H319: Causes serious eye irritation P305+351+338: IF IN EYES: Rinse cautiously with H336: May cause drowsiness or dizziness water for several minutes. Remove contact lenses if present and easy to do – continue rinsing. P337+313: Get medical advice/attention. P403: Store in a well ventilated place. Supplemental Hazard Information (EU) EUH066: Repeated exposure may cause skin dryness or cracking 2.3. Other hazards N/A SAFETY DATA SHEET Acetone Revision date: 12.05.15 SDS/004/8 Page 2 of 6 3. -
Toxicological Profile for Acetone Draft for Public Comment
ACETONE 1 Toxicological Profile for Acetone Draft for Public Comment July 2021 ***DRAFT FOR PUBLIC COMMENT*** ACETONE ii DISCLAIMER Use of trade names is for identification only and does not imply endorsement by the Agency for Toxic Substances and Disease Registry, the Public Health Service, or the U.S. Department of Health and Human Services. This information is distributed solely for the purpose of pre dissemination public comment under applicable information quality guidelines. It has not been formally disseminated by the Agency for Toxic Substances and Disease Registry. It does not represent and should not be construed to represent any agency determination or policy. ***DRAFT FOR PUBLIC COMMENT*** ACETONE iii FOREWORD This toxicological profile is prepared in accordance with guidelines developed by the Agency for Toxic Substances and Disease Registry (ATSDR) and the Environmental Protection Agency (EPA). The original guidelines were published in the Federal Register on April 17, 1987. Each profile will be revised and republished as necessary. The ATSDR toxicological profile succinctly characterizes the toxicologic and adverse health effects information for these toxic substances described therein. Each peer-reviewed profile identifies and reviews the key literature that describes a substance's toxicologic properties. Other pertinent literature is also presented, but is described in less detail than the key studies. The profile is not intended to be an exhaustive document; however, more comprehensive sources of specialty information are referenced. The focus of the profiles is on health and toxicologic information; therefore, each toxicological profile begins with a relevance to public health discussion which would allow a public health professional to make a real-time determination of whether the presence of a particular substance in the environment poses a potential threat to human health. -
Plasma-Pd/Γ-Al203 Catalytic System for Methane, Toluene and Propene Oxidation: Effect of Temperature and Plasma Input Power
22nd International Symposium on Plasma Chemistry July 5-10, 2015; Antwerp, Belgium Plasma-Pd/γ-Al203 catalytic system for methane, toluene and propene oxidation: effect of temperature and plasma input power T. Pham Huu1, 2, P. Da Costa3, S. Loganathan1 and A. Khacef1 1 GREMI-UMR 6744, CNRS-Université d'Orléans, 14 rue d’Issoudun, P.O. Box 6744, FR-45067 Orléans Cedex 02, France 2 Institute of Applied Material Science, Vietnam Academy of Science and Technology, 1 Mac Dinh Chi, HCMC, Vietnam 3 Sorbonne Universités, UPMC Paris 6, Institut Jean Le Rond d’Alembert, CNRS UMR 7190, 2 place de la gare de ceinture, FR-78210 Saint Cyr l’école, France Abstract: A pulsed non-thermal plasma and 1 wt% Pd/γ-Al2O3 catalyst was used to investigate the CH4, C3H6, and C7H8 oxidation in air. Effects of temperature and specific input energy on the VOCs conversion were studied. The plasma-catalyst interaction revealed the benefit effect on the VOCs oxidation even at low temperature leading to high CO2 selectivity. The synergistic effect of combining plasma with catalyst in one-stage configuration is observed only for toluene. Keywords: non-thermal plasma, Pd/γ-Al203, synergistic effect, methane, propene, toluene, oxidation 1. Introduction 2. Experimental Volatile organic compounds (VOCs) emitted from The plasma reactor is a cylindrical DBD gives the various industrial and domestic processes are important possibility to combine the catalyst with plasma reactor in sources of air pollution and therefore, become a serious single stage (IPC) or two-stage (PPC) configuration as problem for damaging the human health and the shown in Fig. -
Annexes To: CPMP/ICH/283/95 Impurities: Guideline for Residual Solvents & CVMP/VICH/502/99 Guideline on Impurities
20 February 2013 CPMP/QWP/450/03 -Rev.1, EMEA/CVMP/511/03 -Rev.1 Committee for medicinal products for human use (CHMP) Committee for medicinal products for veterinary use (CVMP) Annexes to: CPMP/ICH/283/95 Impurities: Guideline for residual solvents & CVMP/VICH/502/99 Guideline on impurities: residual solvents Annex I: specifications for class 1 and class 2 residual solvents in active substances Annex II: residues of solvents used in the manufacture of finished products Discussion at Quality Working Party January 2003 to June 2004 Adoption by CVMP July 2004 Adoption by CHMP July 2004 Date for coming into operation January 2005 Rev. 01 Adoption by Quality Working Party 22 November 2012 Rev. 01 Adoption by CVMP 7 February 2013 Rev. 01 Adoption by CHMP 11 February 2013 Rev. 01 Date for coming into operation 1 March 2013 7 Westferry Circus ● Canary Wharf ● London E14 4HB ● United Kingdom Telephone +44 (0)20 7418 8400 Facsimile +44 (0)20 7418 8416 E -mail [email protected] Website www.ema.europa.eu An agency of the European Union © European Medicines Agency, 2013. Reproduction is authorised provided the source is acknowledged. Annexes to: CPMP/ICH/283/95 Impurities: Guideline for residual solvents & CVMP/VICH/502/99 Guideline on impurities: residual solvents Introduction The two (V)ICH residual solvents guidelines, ICH Q3C Impurities: Guideline for residual solvents (CPMP/ICH/283/95) and VICH GL18 Guideline on impurities: residual solvents in new veterinary medicinal products, active substances and excipients (CVMP/VICH/502/99), have been in operation for several years, since March 1998 and June 2001 respectively. -
Acetone - Toxfaqs™
Acetone - ToxFAQs™ What is acetone? Acetone is a (manmade) chemical that can also be found in the environment. It is a colorless liquid with a distinct smell and taste. It evaporates easily in air, is flammable, and dissolves in water. Acetone is used by humans to dissolve other substances and to produce plastics, paints and coatings, cleaning products, and personal care products. Other manmade sources of acetone are vehicle exhaust, tobacco smoke, and landfills. Acetone is also released naturally by plants, trees, insects, microbes (germs), volcanic erruptions, and forest fires, and can be found naturally in many fruits and vegetables. Low levels of acetone are produced naturally by the human body, and some health conditions can cause these levels to increase. What happens to acetone in the environment? • Most acetone in the environment exists as vapor in the air, and it can travel long distances this way. • About half of the total acetone in air is broken down by sunlight or other chemicals within 22 days. • Acetone moves from the air into water and soil by rain and snow, and moves quickly from water and soil back into the air. It does not bind to soil or build up in animals. • Acetone can enter surface water as manufacturing waste and seep into groundwater from landfills. • Acetone is broken down by microbes or chemicals in water and soil. How can I be exposed to acetone? A strong scent of acetone • Low levels of acetone are in the air, so most people are exposed and irritation in your eyes, to very small amounts through breathing, but these are rarely at nose, and throat are levels that are harmful to your health. -
Extractive Distillation of Acetone-Methanol Mixture Using Dimethyl Sulfoxide As Entrainer
EXTRACTIVE DISTILLATION OF ACETONE-METHANOL MIXTURE USING DIMETHYL SULFOXIDE AS ENTRAINER K PRABHU TEJA NATIONAL INSTITUTE OF TECHNOLOGY SURATHKAL E mail:[email protected] BACKGROUND: Acetone is used as a direct solvent and as a pioneer to the production of Methyl Methacrylate (MMA), Methacrylic Acid, Bisphenol-A, aldol chemicals to name among many. The use of MMA in LCD, Polycarbonate dental fillings from Bisphenol play an indirect role of demand for acetone in consumer electronics and industrial chemicals. Acetone and methanol have very similar normal boiling points (329.2 and 337.5K) and form a homogeneous minimum- boiling azeotrope at 1atm with a composition77.6mol% acetone at 328K. Three solvents are explored that have different normal boiling points (373K for water, 464K for DMSO, and 405K for chloro-benzene). The rst and second solvents drive the acetone overhead while chloro-benzene drives the methanol overhead in the extractive column. fi PROCESS OVERVIEW: 540 kmol/hr of an equal-molar Acetone -Methanol mixture is fed to 24th stage of a 36th staged extractive distillation column. The entrainer from the recovery column along with the makeup stream is fed to the 4th stage giving an overhead pure acetone. The DMSO, heavy key(methanol) along with traces of acetone is fed to 8th stage of a 16th staged entrainer recovery column. High purity methanol is obtained at the overhead with pure DMSO at the bottom which is recycled back to the extraction column. RESULTS: The extractive column has three design degrees of freedom once the total stages and feed locations are fixed: 3..Reflux Reboiler ratio heat input. -
Me2si(Benz[E]Indenyl)
Propene Polymerization Using Homogeneous MAO-Activated Metallocene Catalysts: Me2Si(Benz[e]lndenyI)2ZrClu'MAO vs. Me2Si(2-Me-Benz[e]lndenyI)2ZrClu'MAO STEPHAN JUNGLlNG,t ROLF MULHAUPT,b UDO STEHLlNG/ HANS-HERBERT BRINTZINGER/ DAVID FISCHER/ and FRANZ LANGHAUSER3 'Institut fOr Makromolekulare Chemie and Freiburger Materialforschungszentrum der Albert-Ludwigs-Universitat Freiburg, 0-79104 Freiburg, Germany; 2Fakultat fOr Chemie, Universitat Konstanz, 0-78434 Konstanz, Germany; and 3BASF AG Abteilung ZKP, 0-67056 Ludwigshafen, Germany SYNOPSIS Propene was polymerized at 40°C and 2-bar propene in toluene using methylalumoxane (MAO) activated rac-Me2Si(Benz[elIndenyl)2ZrCI2 (BI) and rac-Me2Si(2-Me Benz[elIndenyl)2ZrCl2 (MBI). Catalyst BI/MAO polymerizes propene with high activity to afford low molecular weight polypropylene, whereas MBI/MAO is less active and produces high molecular weight polypropylene. Variation of reaction conditions such as propene concentration, temperature, concentration of catalyst components, and addition of hydrogen reveals that the lower molecular weight polypropylene produced with BI/MAO results from chain transfer to propene monomer following a 2,1-insertion. A large fraction of both me tallocene catalyst systems is deactivated upon 2,1-insertion. Such dormant sites can be reactivated by H2-addition, which affords active metallocene hydrides. This effect of H2- addition is reflected by a decreasing content of head-to-head enchainment and the formation of polypropylene with n-butyl end groups. Both catalysts show a strong dependence of activity on propene concentration that indicates a formal reaction order of 1. 7 with respect to propene. MBI/MAO shows a much higher dependence of the activity on temperature than BI/MAO. -
Incompatible Chemical Groups.Pdf
Incompatible Chemical Hazard Groups (and some common examples) Mineral Acids Do NOT Store with… Hydrochloric acid Hydrogen peroxide Acetone Sulfuric Acid Sodium hydroxide Methanol Phosphoric Acid Calcium hydroxide Nitric Acid (keep separate) Chloroform Acetic Acid Strong Organic Acids Do NOT Store with… Acetic Acid3, 4 Hydrogen peroxide Acetone Acetonitrile Formic Acid Sodium hydroxide Methanol Benzene Sulfuric Acid Chloroform Special 1. Organic acids are varied and may be incompatible with each other. Notes: Check MSDSs for specifics 2. Store nitric acid separately in its own secondary container. It is a strong oxidizer. 3. Store acetic acid away from oxidizing agents — especially nitric acid. 4. Acetic acid may be stored with some inorganic acids and most flammable solvents but keep in a separate secondary container. (>70% acetic acid is combustible). Weak These are typically not corrosive and not strongly reactive and can be Organic Acids stored with general liquid lab chemicals. Examples include butyric, maleic, and benzoic acids. Non-Flammable Do NOT Store with… Chlorinated Solvents Methylene chloride Acetone Hexane Chloroform Methanol Nitric Acid Trichloroethane Ethanol Hydrogen Peroxide Carbon tetrachloride Organic Solvents Do NOT Store with… Acetone Hydrogen peroxide Nitric Acid Methanol Sodium hydroxide Chromic Acid Phenol Calcium hydroxide Sulfuric Acid Xylene Trichlorfluoromethane Hydrochloric Acid Oxidizers Do NOT Store with… Nitric Acid Sodium metal Paper and oily rags Hydrogen peroxide Isopropyl Alcohol Xylene Chromic Acid Acetone Sodium nitrate Perchloric Acid Ethyl ether Bromate salts . -
Ethene, Propene, Butene and Isoprene Emissions from a Ponderosa Pine Forest Measured by Relaxed Eddy Accumulation
Atmos. Chem. Phys., 17, 13417–13438, 2017 https://doi.org/10.5194/acp-17-13417-2017 © Author(s) 2017. This work is distributed under the Creative Commons Attribution 3.0 License. Ethene, propene, butene and isoprene emissions from a ponderosa pine forest measured by relaxed eddy accumulation Robert C. Rhew1, Malte Julian Deventer1,*, Andrew A. Turnipseed2,*, Carsten Warneke3,4, John Ortega5, Steve Shen1, Luis Martinez6, Abigail Koss3,4, Brian M. Lerner3,4,a, Jessica B. Gilman3,4, James N. Smith5,b, Alex B. Guenther7, and Joost A. de Gouw3 1Department of Geography and Berkeley Atmospheric Sciences Center, University of California Berkeley, Berkeley, CA 94720-4740, USA 22B Technologies, Boulder CO 80301, USA 3Cooperative Institute for Research in Environmental Sciences (CIRES), University of Colorado Boulder, Boulder CO 80309, USA 4NOAA Earth System Research Laboratory, Boulder, CO 80305, USA 5Atmospheric Chemistry Observations and Modeling (ACOM), National Center for Atmospheric Research, Boulder, CO 80301, USA 6Ernest F. Hollings Undergraduate Scholarship program, NOAA, Boulder, CO 80305, USA 7Department of Earth System Science, University of California Irvine, Irvine, CA 92697-3100, USA anow at: Aerodyne Research Inc., Billerica, MA 01821-3976, USA bnow at: Department of Chemistry, University of California Irvine, Irvine, CA 92697-2025, USA *These authors contributed equally to this work. Correspondence to: Robert C. Rhew ([email protected]) Received: 21 April 2017 – Discussion started: 4 May 2017 Revised: 9 September 2017 – Accepted: 28 September 2017 – Published: 10 November 2017 Abstract. Alkenes are reactive hydrocarbons that influence tober flux, based on measurements and modeling, averaged local and regional atmospheric chemistry by playing impor- 62, 52, 24 and 18 µg m−2 h−1 for ethene, propene, butene tant roles in the photochemical production of tropospheric and isoprene, respectively.