Regulation of GABAA Receptor Subunit Expression in Substance Use Disorders
Total Page:16
File Type:pdf, Size:1020Kb
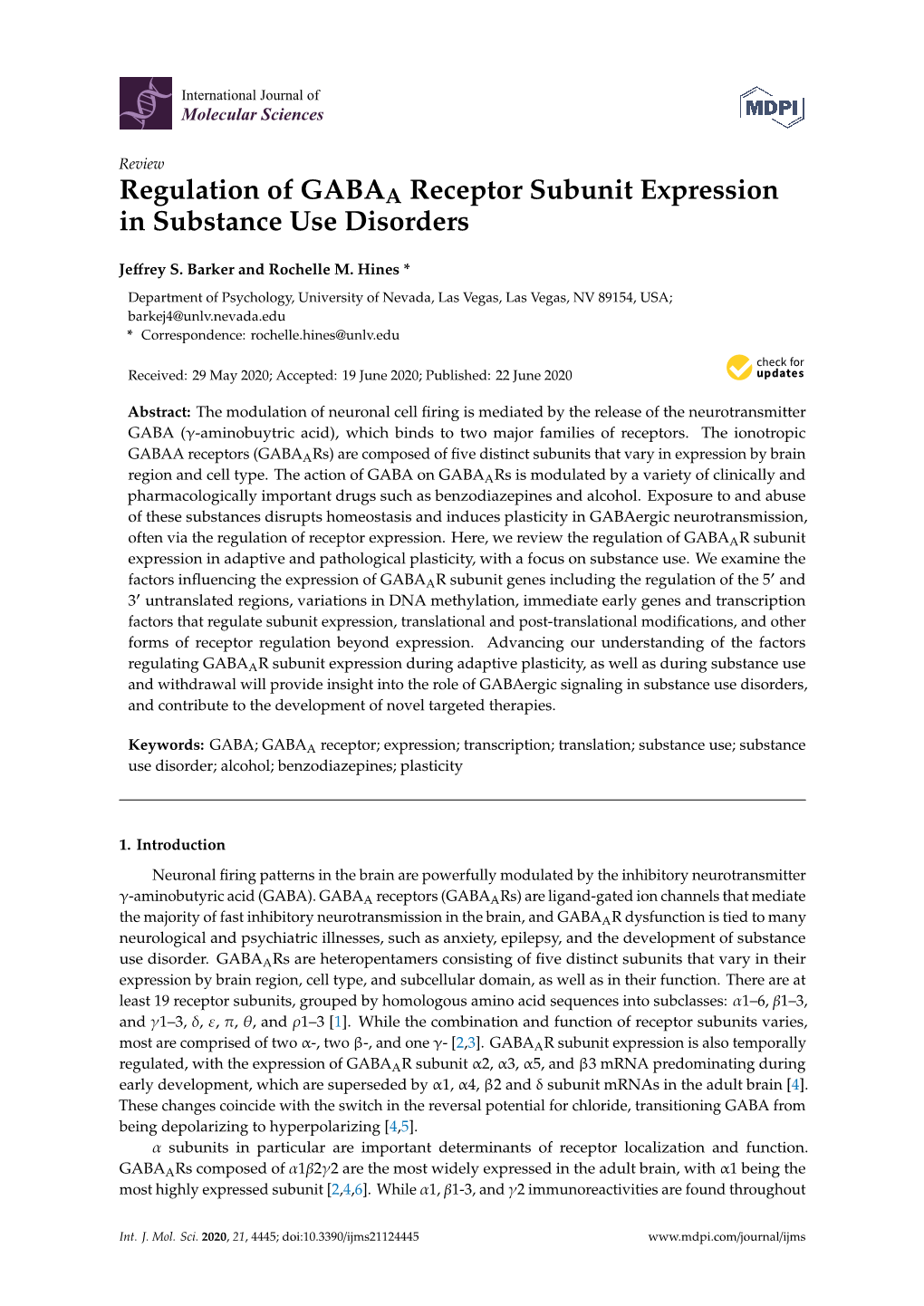
Load more
Recommended publications
-
Sex Differences in a Transgenic Rat Model of Huntington's Disease
Human Molecular Genetics, 2008, Vol. 17, No. 17 2595–2609 doi:10.1093/hmg/ddn159 Advance Access published on May 23, 2008 Sex differences in a transgenic rat model of Huntington’s disease: decreased 17b-estradiol levels correlate with reduced numbers of DARPP321 neurons in males 1,{ 1,{ 1 1 2 Felix J. Bode , Michael Stephan , Hendrik Suhling , Reinhard Pabst , Rainer H. Straub , Downloaded from https://academic.oup.com/hmg/article-abstract/17/17/2595/632381 by guest on 27 June 2020 Kerstin A. Raber3, Michael Bonin4, Huu Phuc Nguyen4, Olaf Riess4, Andreas Bauer5,6, Charlotte Sjoberg7,A˚ sa Peterse´n7 and Stephan von Ho¨ rsten1,3,Ã 1Institute of Functional and Applied Anatomy, Medical School of Hannover, 30625 Hannover, Germany, 2Department of Internal Medicine I, University Regensburg, 93042 Regensburg, Germany, 3Experimental Therapy, Franz-Penzoldt- Center, Friedrich-Alexander-University Erlangen-Nu¨rnberg, 91054 Erlangen, Germany, 4Department of Medical Genetics, University of Tu¨bingen, 72076 Tu¨bingen, Germany, 5Institute of Neurosciences and Biophysics, Research Centre Juelich, 52425 Juelich, Germany, 6Department of Neurology, Heinrich-Heine University Duesseldorf, 40001 Duesseldorf, Germany and 7Translational Neuroendocrine Research Unit, Department of Experimental Medical Science, Lund University, 22184 Lund, Sweden Received March 10, 2008; Revised May 8, 2008; Accepted May 21, 2008 Recent clinical studies have highlighted that female sex hormones represent potential neuroprotective mediators against damage caused by acute and chronic brain diseases. This evidence has been confirmed by experimental studies documenting the protective role of female sex hormones both in vitro and in vivo, although these studies did not specifically focus on Huntington’s disease (HD). -
Cognition and Steroidogenesis in the Rhesus Macaque
Cognition and Steroidogenesis in the Rhesus Macaque Krystina G Sorwell A DISSERTATION Presented to the Department of Behavioral Neuroscience and the Oregon Health & Science University School of Medicine in partial fulfillment of the requirements for the degree of Doctor of Philosophy November 2013 School of Medicine Oregon Health & Science University CERTIFICATE OF APPROVAL This is to certify that the PhD dissertation of Krystina Gerette Sorwell has been approved Henryk Urbanski Mentor/Advisor Steven Kohama Member Kathleen Grant Member Cynthia Bethea Member Deb Finn Member 1 For Lily 2 TABLE OF CONTENTS Acknowledgments ......................................................................................................................................................... 4 List of Figures and Tables ............................................................................................................................................. 7 List of Abbreviations ................................................................................................................................................... 10 Abstract........................................................................................................................................................................ 13 Introduction ................................................................................................................................................................. 15 Part A: Central steroidogenesis and cognition ............................................................................................................ -
The Human Carotid Body Expression of Oxygen Sensing and Signaling Genes of Relevance for Anesthesia
PERIOPERATIVE MEDICINE Anesthesiology 2010; 113:1270–9 Copyright © 2010, the American Society of Anesthesiologists, Inc. Lippincott Williams & Wilkins The Human Carotid Body Expression of Oxygen Sensing and Signaling Genes of Relevance for Anesthesia Malin Jonsson Fagerlund, M.D., Ph.D.,* Jessica Kåhlin, M.D.,† Anette Ebberyd, B.M.A.,‡ Gunnar Schulte, Ph.D.,§ Souren Mkrtchian, M.D., Ph.D.,ʈ Lars I. Eriksson, M.D., Ph.D., F.R.C.A.# Downloaded from http://pubs.asahq.org/anesthesiology/article-pdf/113/6/1270/252613/0000542-201012000-00011.pdf by guest on 28 September 2021 ABSTRACT with DNA microarrays, real-time polymerase chain reaction, Background: Hypoxia is a common cause of adverse events and immunohistochemistry. in the postoperative period, where respiratory depression due Results: We found gene expression of the oxygen-sensing ϩ to residual effects of drugs used in anesthesia is an important pathway, heme oxygenase 2, and the K channels TASK ϩ underlying factor. General anesthetics and neuromuscular (TWIK-related acid sensitive K channel)-1 and BK (large- blocking agents reduce the human ventilatory response to conductance potassium channel). In addition, we show the hypoxia. Although the carotid body (CB) is the major oxygen expression of critical receptor subunits such as ␥-aminobu- ␣  ␥ sensor in humans, critical oxygen sensing and signaling path- tyric acid A ( 2, 3, and 2), nicotinic acetylcholine recep- ␣ ␣  ways have been investigated only in animals so far. Thus, the tors ( 3, 7, and 2), purinoceptors (A2A and P2X2), and aim of this study was to characterize the expression of key the dopamine D2 receptor. genes and localization of their products involved in the hu- Conclusions: In unique samples of the human CB, we here man oxygen sensing and signaling pathways with a focus on demonstrate presence of critical proteins in the oxygen-sens- receptor systems and ion channels of relevance in anesthesia. -
Structural Basis for Potentiation by Alcohols and Anaesthetics in a Ligand-Gated Ion Channel
ARTICLE Received 10 Jul 2012 | Accepted 28 Feb 2013 | Published 16 Apr 2013 DOI: 10.1038/ncomms2682 Structural basis for potentiation by alcohols and anaesthetics in a ligand-gated ion channel Ludovic Sauguet1,2,3,4,*, Rebecca J. Howard5,w,*, Laurie Malherbe1,2,3,4,UiS.Lee5, Pierre-Jean Corringer3,4, R. Adron Harris5 & Marc Delarue1,2 Ethanol alters nerve signalling by interacting with proteins in the central nervous system, particularly pentameric ligand-gated ion channels. A recent series of mutagenesis experi- ments on Gloeobacter violaceus ligand-gated ion channel, a prokaryotic member of this family, identified a single-site variant that is potentiated by pharmacologically relevant concentra- tions of ethanol. Here we determine crystal structures of the ethanol-sensitized variant in the absence and presence of ethanol and related modulators, which bind in a transmembrane cavity between channel subunits and may stabilize the open form of the channel. Structural and mutagenesis studies defined overlapping mechanisms of potentiation by alcohols and anaesthetics via the inter-subunit cavity. Furthermore, homology modelling show this cavity to be conserved in human ethanol-sensitive glycine and GABA(A) receptors, and to involve residues previously shown to influence alcohol and anaesthetic action on these proteins. These results suggest a common structural basis for ethanol potentiation of an important class of targets for neurological actions of ethanol. 1 Unite´ de Dynamique Structurale des Macromole´cules, Institut Pasteur, F-75015 Paris, France. 2 UMR 3258, Centre National de la Recherche Scientifique, F-75015 Paris, France. 3 Groupe Re´cepteurs-Canaux, Institut Pasteur, F-75015 Paris, France. 4 Unite´ de Recherche Associe´e 2182, Centre National de la Recherche Scientifique, F-75015 Paris, France. -
Editing Modifies the GABAA Receptor Subunit A3
Downloaded from rnajournal.cshlp.org on September 29, 2021 - Published by Cold Spring Harbor Laboratory Press REPORT Editing modifies the GABAA receptor subunit a3 JOHAN OHLSON,1 JAKOB SKOU PEDERSEN,2 DAVID HAUSSLER,2,3 and MARIE O¨ HMAN1 1Department of Molecular Biology and Functional Genomics, Stockholm University, SE-106 91 Stockholm, Sweden 2Center for Biomolecular Science and Engineering, University of California Santa Cruz, Santa Cruz, California 95064, USA 3Howard Hughes Medical Institute, University of California at Santa Cruz, Santa Cruz, California 95064, USA ABSTRACT Adenosine to inosine (A-to-I) pre-mRNA editing by the ADAR enzyme family has the potential to increase the variety of the proteome. This editing by adenosine deamination is essential in mammals for a functional brain. To detect novel substrates for A-to-I editing we have used an experimental method to find selectively edited sites and combined it with bioinformatic techniques that find stem–loop structures suitable for editing. We present here the first verified editing candidate detected by this screening procedure. We show that Gabra-3, which codes for the a3 subunit of the GABAA receptor, is a substrate for editing by both ADAR1 and ADAR2. Editing of the Gabra-3 mRNA recodes an isoleucine to a methionine. The extent of editing is low at birth but increases with age, reaching close to 100% in the adult brain. We therefore propose that editing of the Gabra-3 mRNA is important for normal brain development. Keywords: RNA editing; adenosine deaminase; ADAR; GABA receptor; Gabra-3 INTRODUCTION At this site a CAG, coding for glutamine, is modified to CIG, which is read as an arginine codon (CGG). -
Α5gabaa Receptor Activity Sets the Threshold for Long-Term Potentiation and Constrains Hippocampus-Dependent Memory
The Journal of Neuroscience, April 14, 2010 • 30(15):5269–5282 • 5269 Cellular/Molecular ␣ 5GABAA Receptor Activity Sets the Threshold for Long-Term Potentiation and Constrains Hippocampus-Dependent Memory Loren J. Martin,1 Agnieszka A. Zurek,2 John F. MacDonald,2 John C. Roder,3 Michael F. Jackson,2 and Beverley A. Orser1,2,4 1Institute of Medical Science and 2Department of Physiology, University of Toronto, Toronto, Ontario M5S 1A8, Canada, 3Samuel Lunenfeld Research Institute, Mount Sinai Hospital, Toronto, Ontario M5G 1X5, Canada, and 4Department of Anesthesia, Sunnybrook Health Sciences Centre, Toronto, Ontario M4N 3M5, Canada Synaptic plasticity, which is the neuronal substrate for many forms of hippocampus-dependent learning, is attenuated by GABA type A receptor (GABAAR)-mediated inhibition. The prevailing notion is that a synaptic or phasic form of GABAergic inhibition regulates synaptic plasticity; however, little is known about the role of GABAAR subtypes that generate a tonic or persistent inhibitory conductance. ␣ ␣ We studied the regulation of synaptic plasticity by 5 subunit-containing GABAARs ( 5GABAARs), which generate a tonic inhibitory conductance in CA1 pyramidal neurons using electrophysiological recordings of field and whole-cell potentials in hippocampal slices ؊/؊ ␣ from both wild-type and null mutant mice for the 5 subunit of the GABAAR(Gabra5 mice). In addition, the strength of fear- ␣ associated memory was studied. The results showed that 5GABAAR activity raises the threshold for induction of long-term potentiation in a highly specific band of stimulation frequencies (10–20 Hz) through mechanisms that are predominantly independent of inhibitory ␣ synaptic transmission. The deletion or pharmacological inhibition of 5GABAARs caused no change in baseline membrane potential or inputresistancebutincreaseddepolarizationduring10Hzstimulation.Theencodingofhippocampus-dependentmemorywasregulated ␣ by 5GABAARs but only under specific conditions that generate moderate but not robust forms of fear-associated learning. -
Rare GABRA3 Variants Are Associated with Epileptic Seizures, Encephalopathy and Dysmorphic Features
doi:10.1093/brain/awx236 BRAIN 2017: 140; 2879–2894 | 2879 Rare GABRA3 variants are associated with epileptic seizures, encephalopathy and dysmorphic features Cristina Elena Niturad,1,* Dorit Lev,2,3,4,* Vera M. Kalscheuer,5,6,* Agnieszka Charzewska,7 Julian Schubert,1,8 Tally Lerman-Sagie,3,4,9 Hester Y. Kroes,10 Renske Oegema,10 Monica Traverso,11 Nicola Specchio,12 Maria Lassota,13 Jamel Chelly,14 Odeya Bennett-Back,15 Nirit Carmi,3,4,10 Tal Koffler-Brill,16 Michele Iacomino,11 Marina Trivisano,12 Giuseppe Capovilla,17 Pasquale Striano,18 Magdalena Nawara,7 Sylwia Rzon´ca,7 Ute Fischer,5,6 Melanie Bienek,5 Corinna Jensen,5,z Hao Hu,5,§ Holger Thiele,19 Janine Altmu¨ller,19,20 Roland Krause,8 Patrick May,8 Felicitas Becker,1 EuroEPINOMICS Consortium, Rudi Balling,8 Saskia Biskup,21 Stefan A. Haas,22 Peter Nu¨rnberg,19 Koen L. I. van Gassen,10 Holger Lerche,1 Federico Zara,11,* Snezana Maljevic1,*,f and Esther Leshinsky-Silver2,3,16,*,† *These authors contributed equally to this work. †Deceased. Genetic epilepsies are caused by mutations in a range of different genes, many of them encoding ion channels, receptors or transporters. While the number of detected variants and genes increased dramatically in the recent years, pleiotropic effects have also been recognized, revealing that clinical syndromes with various degrees of severity arise from a single gene, a single mutation, or from different mutations showing similar functional defects. Accordingly, several genes coding for GABAA receptor subunits have been linked to a spectrum of benign to severe epileptic disorders and it was shown that a loss of function presents the major correlated pathomechanism. -
Neonatal Clonazepam Administration Induced Long-Lasting Changes in GABAA and GABAB Receptors
International Journal of Molecular Sciences Article Neonatal Clonazepam Administration Induced Long-Lasting Changes in GABAA and GABAB Receptors Hana Kubová 1,* , Zde ˇnkaBendová 2,3 , Simona Moravcová 2,3 , Dominika Paˇcesová 2,3, Luisa Rocha 4 and Pavel Mareš 1 1 Institute of Physiology, Academy of Sciences of the Czech Republic, 14220 Prague, Czech Republic; [email protected] 2 Faculty of Science, Charles University, 12800 Prague, Czech Republic; [email protected] (Z.B.); [email protected] (S.M.); [email protected] (D.P.) 3 National Institute of Mental Health, 25067 Klecany, Czech Republic 4 Pharmacobiology Department, Center of Research and Advanced Studies, Mexico City 14330, Mexico; [email protected] * Correspondence: [email protected]; Tel.: +420-2-4106-2565 Received: 31 March 2020; Accepted: 28 April 2020; Published: 30 April 2020 Abstract: Benzodiazepines (BZDs) are widely used in patients of all ages. Unlike adults, neonatal animals treated with BZDs exhibit a variety of behavioral deficits later in life; however, the mechanisms underlying these deficits are poorly understood. This study aims to examine whether administration of clonazepam (CZP; 1 mg/kg/day) in 7–11-day-old rats affects Gama aminobutyric acid (GABA)ergic receptors in both the short and long terms. Using RT-PCR and quantitative autoradiography, we examined the expression of the selected GABAA receptor subunits (α1, α2, α4, γ2, and δ) and the GABAB B2 subunit, and GABAA, benzodiazepine, and GABAB receptor binding 48 h, 1 week, and 2 months after treatment discontinuation. Within one week after CZP cessation, the expression of the α2 subunit was upregulated, whereas that of the δ subunit was downregulated in both the hippocampus and cortex. -
Gene Expression Profile in Different Age Groups and Its Association With
cells Article Gene Expression Profile in Different Age Groups and Its Association with Cognitive Function in Healthy Malay Adults in Malaysia Nur Fathiah Abdul Sani 1 , Ahmad Imran Zaydi Amir Hamzah 1, Zulzikry Hafiz Abu Bakar 1 , Yasmin Anum Mohd Yusof 2, Suzana Makpol 1 , Wan Zurinah Wan Ngah 1 and Hanafi Ahmad Damanhuri 1,* 1 Department of Biochemistry, Faculty of Medicine, Universiti Kebangsaan Malaysia Medical Center, Jalan Yaacob Latif, Cheras, Kuala Lumpur 56000, Malaysia; [email protected] (N.F.A.S.); [email protected] (A.I.Z.A.H.); zulzikryhafi[email protected] (Z.H.A.B.); [email protected] (S.M.); [email protected] (W.Z.W.N.) 2 Faculty of Medicine and Defence Health, National Defence University of Malaysia, Kem Sungai Besi, Kuala Lumpur 57000, Malaysia; [email protected] * Correspondence: hanafi[email protected] Abstract: The mechanism of cognitive aging at the molecular level is complex and not well under- stood. Growing evidence suggests that cognitive differences might also be caused by ethnicity. Thus, this study aims to determine the gene expression changes associated with age-related cognitive decline among Malay adults in Malaysia. A cross-sectional study was conducted on 160 healthy Malay subjects, aged between 28 and 79, and recruited around Selangor and Klang Valley, Malaysia. Citation: Abdul Sani, N.F.; Amir Gene expression analysis was performed using a HumanHT-12v4.0 Expression BeadChip microarray Hamzah, A.I.Z.; Abu Bakar, Z.H.; kit. The top 20 differentially expressed genes at p < 0.05 and fold change (FC) = 1.2 showed that Mohd Yusof, Y.A.; Makpol, S.; Wan PAFAH1B3, HIST1H1E, KCNA3, TM7SF2, RGS1, and TGFBRAP1 were regulated with increased Ngah, W.Z.; Damanhuri, H.A. -
The Role of GABRA2 in Risk for Conduct Disorder and Alcohol and Drug Dependence Across Developmental Stages
Behavior Genetics, Vol. 36, No. 4, July 2006 (Ó 2006) DOI: 10.1007/s10519-005-9041-8 The Role of GABRA2 in Risk for Conduct Disorder and Alcohol and Drug Dependence across Developmental Stages Danielle M. Dick,1,9 Laura Bierut,1 Anthony Hinrichs,1 Louis Fox,1 Kathleen K. Bucholz,1 John Kramer,2 Samuel Kuperman,2 Victor Hesselbrock,3 Marc Schuckit,4 Laura Almasy,5 Jay Tischfield,6 Bernice Porjesz,7 Henri Begleiter,7 John Nurnberger Jr.,8 Xiaoling Xuei,8 Howard J. Edenberg,8 and Tatiana Foroud8 Received Apr. 28 2005—Final Dec. 22 2005 We use findings from the behavior genetics literature about how genetic factors (latently) influence alcohol dependence and related disorders to develop and test hypotheses about the risk associated with a specific gene, GABRA2, across different developmental stages. This gene has previously been associated with adult alcohol dependence in the Collaborative Study of the Genetics of Alcoholism (COGA) sample [Edenberg, H. J., Dick, D. M., Xuei, X., Tian, H., Almasy, L., Bauer, L. O., Crowe, R., Goate, A., Hesselbrock, V., Jones, K. A., Kwon, J., Li, T. K., Nurnberger Jr., J. I., OÕConnor, S. J., Reich, T., Rice, J., Schuckit, M., Porjesz, B., Foroud, T., and Begleiter, H. (2004). Am. J. Hum. Genet. 74:705–714] and other studies [Covault, J., Gelernter, J., Hesselbrock, V., Nellissery, M., and Kranzler, H. R. (2004). Am. J. Med. Genet. B Neuropsychiatr. Genet. 129B:104–109; Lappalainen, J., Krupitsky, E., Remizov, M., Pchelina, S., Taraskina, A., Zvartau, E., Somberg, L. K., Covault, J., Kranzler, H. R., Krystal, J., and Gelernter, J. -
Molecular Mechanisms Driving Prostate Cancer Neuroendocrine Differentiation
Molecular mechanisms driving prostate cancer neuroendocrine differentiation Submitted by Joseph Edward Sutton Supervisory team: Dr Amy Poole (DoS) Dr Jennifer Fraser Dr Gary Hutchison A thesis submitted in partial fulfilment of the requirements of Edinburgh Napier University, for the award of Doctor of Philosophy. October 2019 School of Applied Sciences Edinburgh Napier University Edinburgh Declaration It is hereby declared that this thesis is the result of the author’s original research. It has been composed by the author and has not been previously submitted for examination which has led to the award of a degree. Signed: II Dedication This thesis is dedicated to my grandfather William ‘Harry’ Russell, who died of stomach cancer in 2014. Thank you for always encouraging me to achieve my ambitions, believing in me and for retaining your incredible positivity and sense of humour, even at the very end of your life. III Acknowledgements First of all, I would like to acknowledge my parents, who dedicated so much effort and energy into helping me to achieve my lifelong ambition of becoming a scientist. From taking me to the Natural History and Science Museums in London as a child, to tolerating my obsession with Jurassic Park and continuing to support me in both of your unique yet equally important ways, thank you. I would also like to thank my PhD supervisors Dr Amy Poole and Dr Jenny Fraser, not only for their excellent scientific guidance but also for their great banter and encouragement along the way. Thank you for seeing some potential in me, taking a chance on me and for helping me to continue my scientific journey. -
A Human Stem Cell-Derived Test System for Agents Modifying Neuronal N
Archives of Toxicology (2021) 95:1703–1722 https://doi.org/10.1007/s00204-021-03024-0 IN VITRO SYSTEMS A human stem cell‑derived test system for agents modifying neuronal 2+ N‑methyl‑D‑aspartate‑type glutamate receptor Ca ‑signalling Stefanie Klima1,2 · Markus Brüll1 · Anna‑Sophie Spreng1,3 · Ilinca Suciu1,3 · Tjalda Falt1 · Jens C. Schwamborn4 · Tanja Waldmann1 · Christiaan Karreman1 · Marcel Leist1,5 Received: 28 October 2020 / Accepted: 4 March 2021 / Published online: 13 March 2021 © The Author(s) 2021 Abstract Methods to assess neuronal receptor functions are needed in toxicology and for drug development. Human-based test systems that allow studies on glutamate signalling are still scarce. To address this issue, we developed and characterized pluripotent stem cell (PSC)-based neural cultures capable of forming a functional network. Starting from a stably proliferating neu- roepithelial stem cell (NESC) population, we generate “mixed cortical cultures” (MCC) within 24 days. Characterization by immunocytochemistry, gene expression profling and functional tests (multi-electrode arrays) showed that MCC contain various functional neurotransmitter receptors, and in particular, the N-methyl-D-aspartate subtype of ionotropic glutamate receptors (NMDA-R). As this important receptor is found neither on conventional neural cell lines nor on most stem cell- derived neurons, we focused here on the characterization of rapid glutamate-triggered Ca2+ signalling. Changes of the intra- 2+ cellular free calcium ion concentration ([Ca ]i) were measured by fuorescent imaging as the main endpoint, and a method to evaluate and quantify signals in hundreds of cells at the same time was developed. We observed responses to glutamate in the low µM range.